Thank you for visiting nature.com. You are using a browser version with limited support for CSS. To obtain the best experience, we recommend you use a more up to date browser (or turn off compatibility mode in Internet Explorer). In the meantime, to ensure continued support, we are displaying the site without styles and JavaScript.
- View all journals
- My Account Login
- Explore content
- About the journal
- Publish with us
- Sign up for alerts
- Open access
- Published: 11 January 2023

The Stroop effect involves an excitatory–inhibitory fronto-cerebellar loop
- Moe Okayasu ORCID: orcid.org/0000-0003-0478-6170 1 ,
- Tensei Inukai ORCID: orcid.org/0000-0002-6896-2965 1 ,
- Daiki Tanaka ORCID: orcid.org/0000-0002-9385-0703 1 ,
- Kaho Tsumura ORCID: orcid.org/0000-0001-8398-3134 1 ,
- Reiko Shintaki ORCID: orcid.org/0000-0002-9837-9949 1 ,
- Masaki Takeda 2 ,
- Kiyoshi Nakahara ORCID: orcid.org/0000-0001-6701-6216 2 &
- Koji Jimura ORCID: orcid.org/0000-0003-1991-3371 1 , 2 , 3
Nature Communications volume 14 , Article number: 27 ( 2023 ) Cite this article
7563 Accesses
3 Citations
16 Altmetric
Metrics details
- Cognitive control
- Human behaviour
The Stroop effect is a classical, well-known behavioral phenomenon in humans that refers to robust interference between language and color information. It remains unclear, however, when the interference occurs and how it is resolved in the brain. Here we show that the Stroop effect occurs during perception of color–word stimuli and involves a cross-hemispheric, excitatory–inhibitory loop functionally connecting the lateral prefrontal cortex and cerebellum. Participants performed a Stroop task and a non-verbal control task (which we term the Swimmy task), and made a response vocally or manually. The Stroop effect involved the lateral prefrontal cortex in the left hemisphere and the cerebellum in the right hemisphere, independently of the response type; such lateralization was absent during the Swimmy task, however. Moreover, the prefrontal cortex amplified cerebellar activity, whereas the cerebellum suppressed prefrontal activity. This fronto–cerebellar loop may implement language and cognitive systems that enable goal-directed behavior during perceptual conflicts.
Similar content being viewed by others
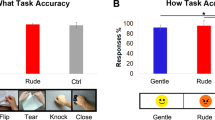
Understanding the attitude of others by hearing action sounds: the role of the insula
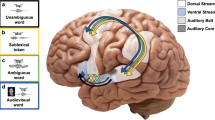
Motor engagement relates to accurate perception of phonemes and audiovisual words, but not auditory words
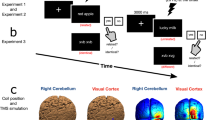
A causal role for the cerebellum in semantic integration: a transcranial magnetic stimulation study
Introduction.
The Stroop effect is widely acknowledged as a robust and intriguing behavioral phenomenon referring to a prolonged reaction time when naming the font color of a printed word if this color differs from that represented by the word’s meaning 1 , 2 , 3 , 4 (Fig. 1a ). It is thought to be attributable to interference between language and color information, and resolution of the interference requires high cognitive control.
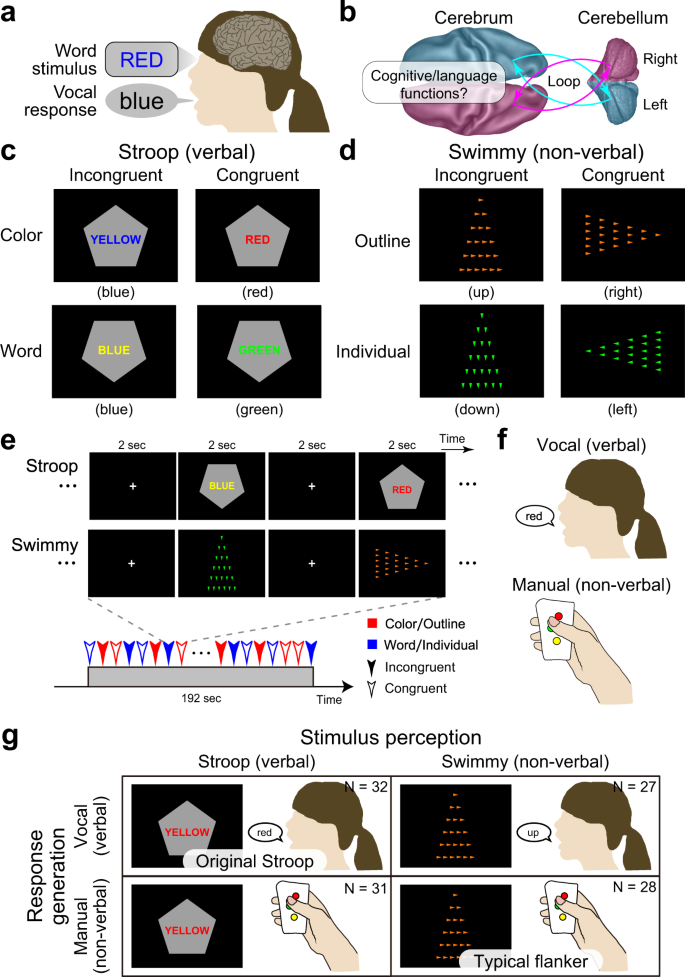
a Stroop effect. It takes longer to name the color of a colored word when the word is colored in an inconsistent color (e.g., the word “RED” in blue-color font). b A schematic illustration of cross-hemispheric cerebro-cerebellar loops. c Stroop tasks. Participants made a judgment regarding the color (top) or word (bottom) of a colored word (Stroop task). The judgment was indicated by the orientation of the vertex of a pentagon (up: color; down: word). The word color and colored word were inconsistent (incongruent; left) or consistent (congruent; right). d Control (Swimmy) tasks. Another set of participants made a judgment regarding the orientation of the vertex of a large outline of a triangle (top) or of small individual (bottom) triangles (Swimmy task). The task dimension as indicated by the color of the triangles (orange: outline; green: individual). The orientation of the outline and individual triangles was inconsistent (incongruent; left) or consistent (congruent; right). e Behavioral procedures. In both the Stroop and Swimmy tasks, four trial conditions (two levels of tasks and two levels of congruency) were presented pseudorandomly. f Participants responded vocally (left) or manually (right). g The experimental conditions configured a 2 × 2 factorial design.
The Stroop effect is possibly unique to humans since it relates to language functions involved in reasoning, problem-solving, and other elaborated processing operations that characterize flexible human behavior 5 , 6 , 7 , 8 , 9 , 10 . Thus, one notable signature of the Stroop effect is that these language functions persistently interfere with color information 2 , 11 , 12 , 13 , 14 , 15 . Despite the importance and long history of this effect, it remains unclear when it occurs and how it is resolved.
The Stroop effect was originally reported based on vocal responses 1 , 13 , 16 , which involve two stages of language processing: perception of a word stimulus and generation of a vocal response (Fig. 1a ). It is well known that distinct regions of the brain are responsible for these two stages 17 , 18 . Behavioral studies have also suggested that the vocal and manual response involve distinct processing during the resolution of the Stroop effect 15 , 19 , 20 . These observations suggest that response generation and its underlying neural mechanisms play an important role in the Stroop effect. However, previous neuroimaging studies of the vocal response 21 , 22 , 23 , 24 , manual response 12 , 14 , 23 , 25 , 26 , 27 , and covert response 23 , 28 , 29 have consistently suggested that the Stroop effect is associated with the anterior cingulate, medial prefrontal, and bilateral prefrontal cortices. Nonetheless, bilateral and medial prefrontal involvement is inconsistent with the traditional view that the human language system is predominantly implicated in the left hemisphere 18 , 30 .
While Stroop tasks induce interference of verbal information processing, cognitive interference occurs in the absence of verbal information as demonstrated by flanker tasks. In a common flanker task, verbal information is not involved in either stimulus perception or response generation 31 , whereas other flanker-type tasks use a verbal stimulus 32 , 33 . Interestingly, as is the case in Stroop tasks, the interference caused by flanker tasks involves the anterior cingulate, bilateral prefrontal, and parietal cortices 32 , 33 , 34 , 35 , 36 , 37 , 38 . This raises a question about whether the resolution of Stroop- and flanker-type non-verbal interference shares core brain mechanisms. If this were the case, such mechanisms would play a generic role in the resolution of cognitive interference beyond the verbality of the stimulus and response 23 ; if not, the resolution would depend on the verbality, suggesting that language processing is specifically involved in the Stroop effect 33 .
It is widely accepted that the brain regions responsible for cognitive and language functions are associative neocortical regions distributed in the cerebrum 17 , 18 , 30 , 39 . Increasing evidence suggests that the cerebellum also plays important roles in language and cognitive control 40 , 41 , 42 , 43 , 44 , 45 , 46 , 47 , 48 , 49 . This cerebellar involvement is associated with dorsal regions in the lateral hemispheres (crus I/II, lobules VI/VIIb) 41 , 45 , 46 , and is independent of sensorimotor functions implicated in rostral and caudal ventromedial regions (lobules I-VI, VIIIa/b) 41 , 50 , 51 . These cerebellar regions constitute a cortico-cerebellar loop between cerebral cortical regions in the contra-lateral hemisphere 41 , 44 , 50 , 51 (Fig. 1b ). Importantly, damage to the dorsolateral cerebellar regions impairs language functions 41 , 45 , 52 , and a classical neuroimaging study reported that language processing without vocal response involves a cerebellar region in the right hemisphere 52 . This collective evidence suggests that a cross-hemispheric cerebro-cerebellar loop is involved in cognitive and language functions (Fig. 1b ). Accordingly, we asked whether the cerebellum plays an important role during the resolution of the Stroop effect involving cognitive and language processing.
In the current study, we examined the role of language processing in the Stroop effect by manipulating the verbality of stimulus perception and response generation involved in the interference resolution. Specifically, we conducted a set of functional MRI experiments where humans performed a Stroop task or a non-verbal control task (which we term the Swimmy task) using vocal or manual responses (Fig. 1c–g ). The Swimmy task served as a control condition in which the stimulus did not involve language information, similar to a flanker task combining the Simon and local–global tasks 53 , 54 . Then, we comprehensively explored language processing during interference in the Stroop and Swimmy tasks (Fig. 1g ), and examined directional effective connectivity between responsible brain regions to identify task-related signal flows between the regions.
Behavioral tasks
A group of human participants ( N = 63) performed a Stroop task. In each trial, a colored word was presented on a gray, pentagon-shaped background, and participants were required to judge the color or word of the stimulus, depending on the orientation of the pentagon vertex (Fig. 1c ). The color and word were either incongruent or congruent.
Another group of participants ( N = 55) performed another task that served as a control of the Stroop task in terms of stimulus verbality, in which the stimulus did not involve verbal information (Fig. 1d ). We named this task the Swimmy task after an old picture book in which small fish were arranged in a pattern that resembled a large fish 55 . In each trial, a set of small, individual triangles was presented on the screen to form the outline of a larger triangle. Participants judged the orientation of the outline or individual triangles (in each case based on the vertex with the smallest angle), depending on the color of the individual triangles. The orientations of the outline and individual triangles were either incongruent or congruent.
In both the Stroop and Swimmy tasks, the task procedures were matched except for the nature of the visual stimulus (Fig. 1e ), and participants responded either vocally (Stroop: N = 32; Swimmy: 27) or manually (Stroop: N = 31; Swimmy: 28) (Fig. 1f ). Thus, the current experimental design consisted of two levels of stimulus modality [verbal (Stroop), non-verbal (Swimmy)] and two levels of response modality (vocal, manual), entailing a 2 × 2 factorial design (Fig. 1g ). In the vocal conditions, responses were recorded through an MRI-compatible noise-reduction microphone (Supplementary Fig. 1 ).
Behavioral performance
In the Stroop task, accuracy was lower in incongruent than congruent trials in both the vocal and manual conditions [vocal: F(1, 31) = 44.0, P < 0.001; manual: F(1, 30) = 48.5, P < 0.001; Fig. 2a left]. In the vocal Stroop task, the interaction effect of congruency (incongruent and congruent) and task (color and word) was also significant [F(1, 31) = 9.0, P < 0.01], but such interaction was absent in the manual condition [F(1, 30) = 1.5, P = 0.23]. Accuracy was lower in the manual than the vocal condition [F(1, 61) = 20.0, P < 0.001]. The interaction effect of response modality (vocal and manual) and congruency was significant [F(1, 61) = 15.6, P < 0.001].
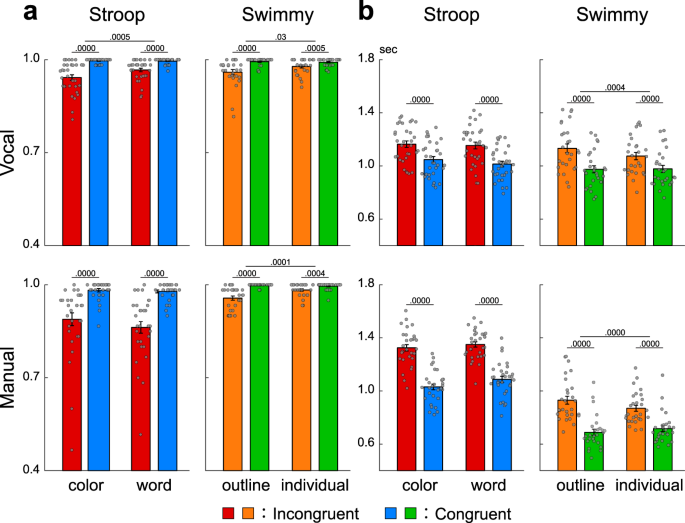
a Accuracy in the Stroop (left) and Swimmy (right) tasks with vocal (top) and manual (bottom) responses (vocal Stroop: N = 32; manual Stroop: N = 31; vocal Swimmy: N = 27; manual Swimmy: N = 28; independent participants). The vertical and horizontal axes indicate accuracy and conditions (task and congruency), respectively. Rectangular and error bars indicate means and standard errors of the mean across participants, respectively, with individual data overlaid on each rectangular bar. Statistical p values are shown on the top (paired t tests, two-tailed, uncorrected). Red: incongruent Stroop; blue: congruent Stroop; orange: incongruent Swimmy; green: congruent Swimmy. b Reaction times. The vertical and horizontal axes indicate reaction times and conditions, respectively. Statistical procedures and formats are similar to those in a . The sample size is identical to that in a .
In the Swimmy task, accuracy was lower in the incongruent than the congruent trials in both the vocal and manual conditions, similar to the Stroop task [vocal: F(1, 26) = 18.1, P < 0.001; manual: F(1, 27) = 40.6, P < 0.001; Fig. 2a right]. In the vocal Swimmy task, the interaction effect of congruency (incongruent and congruent) and task (outline and individual) was also significant [vocal: F(1, 26) = 5.5, P < 0.05; manual: F(1, 27) = 20.0, P < 0.001], indicating that the interference effect was greater in the outline task. There was no significant difference in accuracy between the vocal and manual conditions [F(1, 53) = 0.45, P = 0.51]. The interaction effect of response modality and congruency was not significant [F(1, 53) = 0.17, P = 0.68].
In the vocal conditions, reaction times were calculated as the latency from stimulus onset to vocal response onset (Supplementary Fig. 1 ). In the Stroop task, reaction times were longer in the incongruent than congruent trials in both the vocal and manual conditions [vocal: F(1, 31) = 123.5, P < 0.001; manual: F(1, 30) = 149.3, P < 0.001; Fig. 2b left]. The interaction effect of congruency and task (color and word) was insignificant in both response modalities [vocal: F(1, 31) = 3.6, P = 0.07; manual: F(1, 30) = 4.1, P = 0.05]. Reaction times were slower in the manual than vocal conditions [F(1, 61) = 13.3, P < 0.01]. The interaction effect of response modality and congruency was significant [F(1, 61) = 35.3, P < 0.001].
In this study, reaction times were longer than in the classical Stroop studies 11 because of the pseudorandomized event-related fMRI design in which baseline cognitive demand was high. However, the interference effect (i.e., incongruent vs. congruent) was 117 ± 67 (mean ± SD) ms in the color task and 140 ± 80 ms in the word task in the vocal conditions, and 294 ± 145 ms in the color task and 263 ± 123 ms in the word task in the manual condition, values that were comparable to those in previous studies 15 , 19 , 20 .
In the Swimmy task, reaction times were longer in the incongruent than congruent trials in both the vocal and manual conditions, similar to the Stroop task [vocal: F(1, 26) = 111.0, P < 0.001; manual: F(1, 27) = 196.4, P < 0.001; Fig. 2b right]. The interaction effect of congruency and task was significant in both response modalities [vocal: F(1, 26) = 16.8, P < 0.001; manual: F(1, 27) = 62.1, P < 0.01], which is attributable to greater interference effect in the outline task. Reaction times were longer in the vocal than manual conditions [F(1, 53) = 45.2, P < 0.001]. The interaction effect of response modality and congruency was significant [F(1, 53) = 14.3, P < 0.001].
These collective behavioral results demonstrated that cognitive interference was successfully imposed during the incongruent trials in both the Stroop and Swimmy tasks using vocal and manual responses.
The Stroop effect involves cross-hemispheric cerebro-cerebellar mechanisms
In an imaging analysis, we first explored brain regions associated with the interference effect (i.e., incongruent vs. congruent trials) during the Stroop task (vocal and manual conditions collapsed; N = 63). A strong interference effect was observed in the lateral prefrontal cortex (lPFC), posterior parietal cortex (PPC), and occipitotemporal cortex (OTC) (Fig. 3a and Supplementary Table 1 ; the vocal and manual conditions are collapsed). Interestingly, these prominent activations in cortical regions were observed mainly in the left hemisphere. The cerebellum also showed a strong interference effect, specifically in the crus I/lobule VI (a dorsal and caudal region) and the crus II/lobule VIIb (a dorsal and rostral region) of the cerebellar hemisphere. Notably, this cerebellar involvement was observed mainly in the right hemisphere, which contrasts with the left lateralization in the cortical regions. Involvement of the cortical and cerebellar regions was consistently observed in the vocal and manual conditions when these were analyzed separately (Supplementary Fig. 2 ). The medial prefrontal cortex (mPFC) and anterior cingulate cortex (ACC) also showed interference effects, which is consistent with the fact that these regions are considered to play a key role in the resolution of the Stroop interference 12 , 16 , 23 , 24 , 25 , 29 , 56 , 57 , 58 , 59 , 60 , 61 , 62 , 63 . The activations look weaker compared to those in the left lateral cerebral and right cerebellar regions, however.
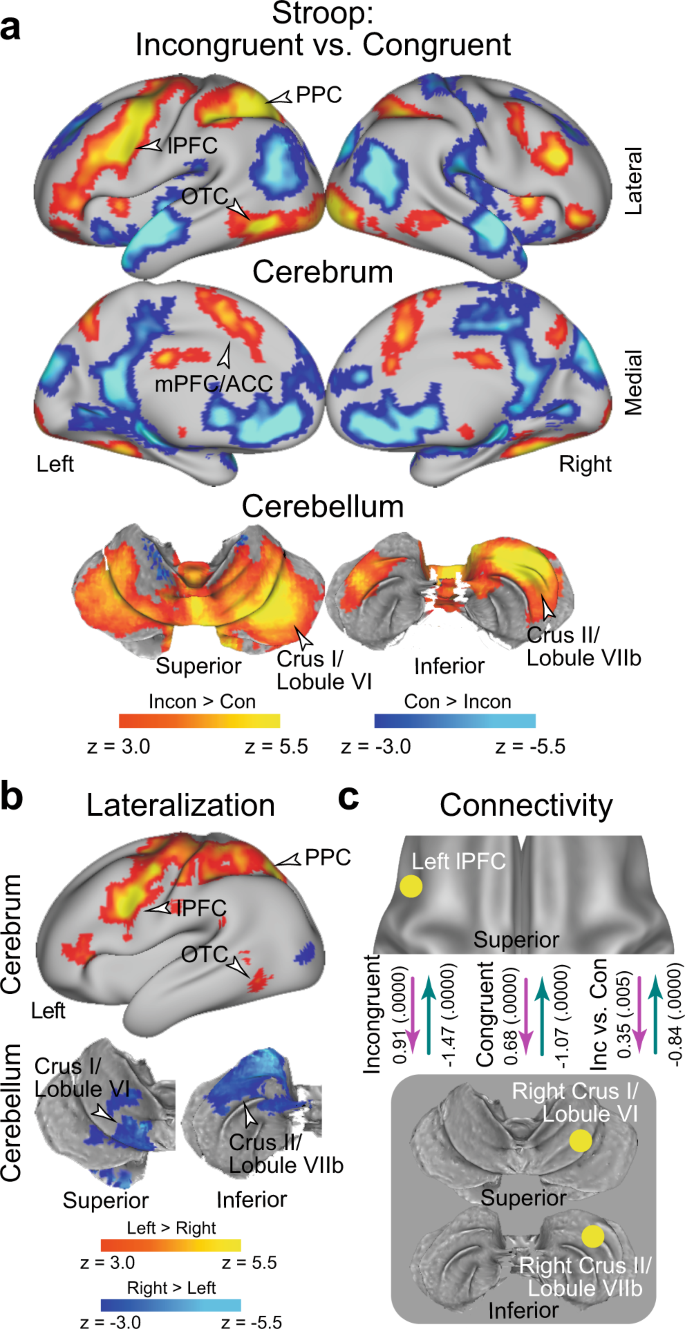
a Statistical activation maps for signal increase and decrease in the contrast between incongruent and congruent trials in the Stroop task ( P < 0.05, FWE-corrected across the whole-brain based on non-parametric permutation tests). The vocal and manual conditions were collapsed. Maps are overlaid onto a 3D surface of the brain. Hot and cool colors indicate signal increase and decrease in the incongruent trials, respectively. Arrowheads indicate anatomical locations of major activations. lPFC: lateral prefrontal cortex; PPC: posterior parietal cortex; OTC: occipitotemporal cortex; mPFC: medial prefrontal cortex; ACC: anterior cingulate cortex. b Statistical maps of brain regions showing differential Stroop effect activation ( a ) between the left and right hemispheres. Hot and cool colors indicate greater activity in the left and right hemispheres, respectively. c Task-related effective connectivity analysis between lPFC and cerebellar regions based on dynamic causal modeling. The values indicate estimates of the connectivity and their p-values calculated based on posterior probability density (one-tailed, uncorrected) are shown in parentheses next. The arrow directions indicate task-related effective connectivity. The magenta and green arrows indicate positive and negative effects, respectively.
Prior behavioral studies have demonstrated asymmetry of interference effects in the color-naming task (i.e., Stroop effect) and word-naming task (i.e., reverse-Stroop effect) 64 , 65 , 66 , 67 . In particular, the interference effects were greater in the color-naming task than in the word-naming task, suggesting stronger cognitive demand to attend to color. Indeed, our behavioral analysis of accuracy in the vocal condition showed a significant interaction effect of congruency and task (color and word), an asymmetric interference pattern that has been observed in reaction times 11 . To test whether the variability in behavioral interference effects is reflected in brain activity, we explored brain regions associated with the interference effect for the color and word tasks separately. In both the color and word tasks, a strong interference effect was observed in the lPFC, PPC, and OTC, all mainly in the left hemisphere, and also in right cerebellar regions; overall, the activation maps look similar for both tasks (Supplementary Fig. 3a/b ). When comparing the neural interference effect between the color and word tasks, differential activity was almost absent (Supplementary Fig. 3c ). Our results suggest that the Stroop and reverse-Stroop effects involve common neural mechanisms, whereas a previous study reported differential effects in cortical regions 67 .
We then asked whether the neocortical and cerebellar regions are lateralized. To this end, we contrasted the activation maps for the interference effect between the left and right hemispheres on a voxel-by-voxel basis, and then explored the brain regions showing a differential interference effect between hemispheres 68 (Supplementary Fig. 4 ; see also Materials and Methods). Greater activity in the left hemisphere was observed in the lPFC, PPC, and OTC. In the cerebellum, on the other hand, the crus I/lobule VI and crus II/lobule VIIb showed greater activity in the right hemisphere (Fig. 3b ). These results clearly demonstrate cross-hemispheric involvement in the neocortex and cerebellum.
Given the cross-hemispheric, lateralized cerebro-cerebellar involvement, we next asked how the cortical and cerebellar regions interacted during the resolution of Stroop interference. To address this issue, we performed an interregional effective connectivity analysis based on dynamic causal modeling (DCM), which makes it possible to examine the directionality of task-related functional connectivity based on the state-space model (see Materials and Methods) 68 , 69 , 70 , 71 , 72 , 73 , 74 . The regions of interest (ROIs) were defined as the left lPFC and the right cerebellar regions (CER; crus I/lobule VI and crus II/lobule VIIb), all of which showed robust activation (Fig. 3a ) and lateralization (Fig. 3b ). To avoid circular analysis 75 , the ROIs were defined independently of the tested data. Specifically, for the analysis of the vocal condition, the lPFC and CER ROIs were defined as the regions showing the interference effect in the manual condition (Supplementary Fig. 2b ), and vice versa (Supplementary Fig. 2a ).
In both the incongruent and congruent trials, the connectivity was excitatory from the lPFC to the CER ( P < 0.001), but inhibitory from the CER to the lPFC ( P < 0.001) (Fig. 3c ). Interestingly, the excitatory connectivity from the lPFC to the CER and the inhibitory connectivity from the CER to the lPFC were strengthened in the incongruent trials relative to the congruent trial ( P < 0.001; Fig. 3c ). The original results were confirmed when the lPFC ROI was defined based on a meta-analysis map of cognitive control 62 (see Methods) (Supplementary Fig. 5 ).
When the crus I/lobule VI and crus II/lobule VIIb were analyzed separately, consistent results were obtained, suggesting that these two cerebellar regions implement homologous functions in the Stroop effect (Supplementary Fig. 6a/b ). Moreover, these connectivity results were observed in the vocal and manual conditions consistently (Supplementary Fig. 6c–h ), suggesting that the functional connectivity between the lPFC and the CER was independent of response modality. On the other hand, the color task showed stronger excitatory connectivity and weaker inhibitory connectivity relative to the word task (Supplementary Fig. 7 ).
The Swimmy effect involves bilateral cerebro-cerebellar mechanisms
In the Swimmy task, a strong interference effect (incongruent vs. congruent trials) was observed bilaterally in the lPFC, PPC, and OTC. In the cerebellum, the crus I/lobule VI (a dorsal and caudal region) and the crus II/lobule VIIb (a dorsal and rostral region) also showed strong bilateral interference effects (Fig. 4a and Supplementary Table 2 ). The involvement of these cortical and cerebellar regions was predominant in both the vocal and manual conditions when these were analyzed separately (Supplementary Fig. 8 ). These results suggest that the resolution of the Swimmy effect involves bilateral cortical and cerebellar regions, independently of response modality. This response modality-independent involvement was consistent with that observed in the Stroop task. The outline and individual tasks involved similar cortical and cerebellar regions (Supplementary Fig. 9a, b ), but the interference effect differed between the two tasks in multiple neocortical and cerebellar regions (Supplementary Fig. 9c ), possibly reflecting distinctive attention to global and local shapes 76 .
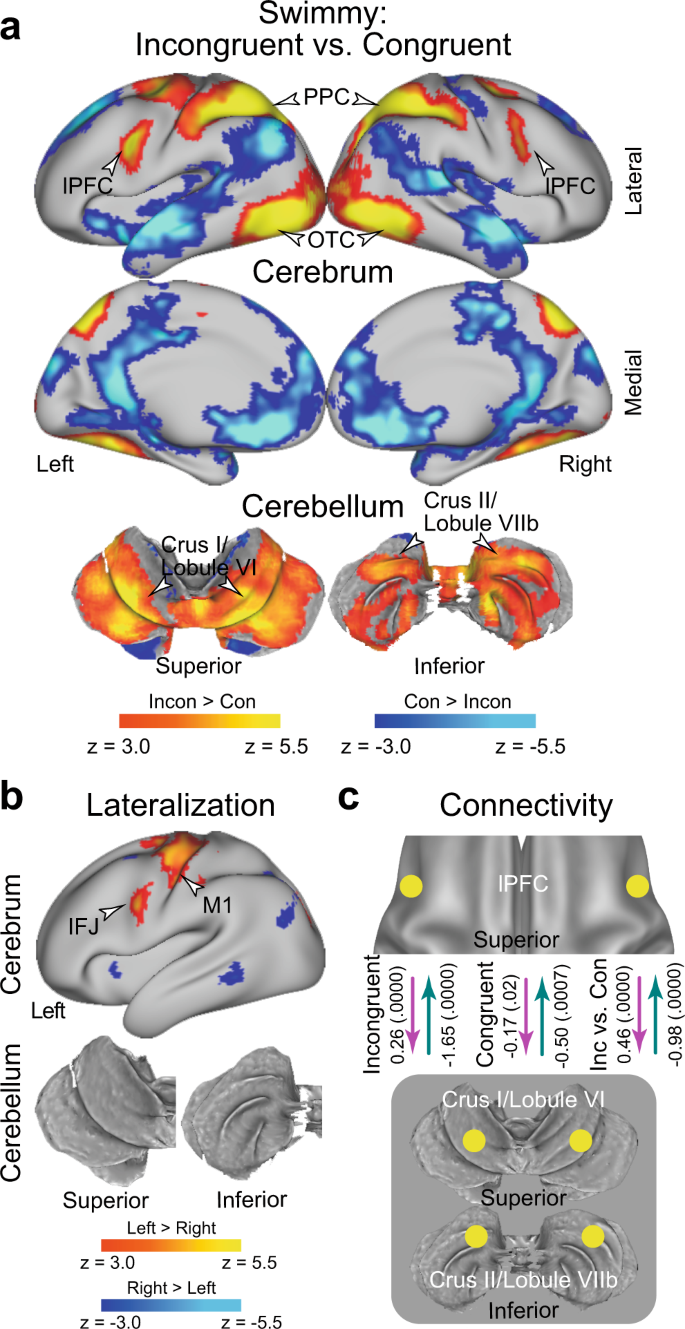
a Statistical activation maps for signal increase and decrease in the contrast between incongruent and congruent trials in the Swimmy task. The vocal and manual conditions were collapsed. b Statistical maps of brain regions showing differential Swimmy effect activation ( a ) between the left and right hemispheres. IFJ inferior frontal junction. c Effective connectivity analysis between the lPFC and cerebellar regions based on dynamic causal modeling. The dashed arrows indicate insignificant connectivity. Statistical procedures and formats are similar to those in Fig. 3 .
We then explored the laterality of activity during the Swimmy interference. The inferior frontal junction (IFJ) and the primary motor cortex showed stronger activity in the left hemisphere (Fig. 4b ); however, compared to the Stroop task (Fig. 3b ), the lateralization was weaker across the neocortical regions. It should be noted that the IFJ region is located in the posterior bank of the precentral sulcus (Fig. 3b ), and is spatially separated from the lPFC region located in the inferior frontal sulcus. In the cerebellum, there was no lateralized activity. These results suggest that relative to the Stroop effect, the Swimmy effect involves neocortical and cerebellar areas more bilaterally.
We next performed DCM analysis of bilateral lPFC and CER ROIs. In the incongruent trials, the connectivity was excitatory from the lPFC to the CER, and inhibitory from the CER to the lPFC (Fig. 4c ) ( P < 0.001). However, connectivity was weak in the congruent trials ( P s > 0.07). The excitatory connectivity from the lPFC to the CER and the inhibitory connectivity from the CER to the lPFC was greater in the incongruent trials than in the congruent trials ( P s < 0.001; Fig. 4c ). The original results were confirmed when the lPFC ROI was defined based on a meta-analysis map of cognitive control 62 (see Methods) (Supplementary Fig. 10 ), similarly to the DCM analysis for the Stroop effect (Supplementary Fig. 5 ).
Again, these results are consistently observed in both the vocal and manual conditions when the two conditions were analyzed separately (Supplementary Fig. 11a/b ). DCM analysis of the lPFC and CER in unilateral and cross-hemispheric ROIs also exhibited excitatory and inhibitory connectivity (Supplementary Fig. 11c–f ). This suggests that in terms of functional involvement and effective connectivity, the left and right hemispheres implement homogeneous functionality during the Swimmy task, in contrast to the Stroop task. Connectivity strength was not robustly differed between the outline and individual tasks (Supplementary Fig. 12 ).
Fronto-cerebellar involvement is independent of response modality
To examine whether response modality (vocal vs. manual) is critical for the interference effect, we directly compared brain activity between the vocal and manual conditions (Supplementary Fig. 13 ). When the incongruent and congruent trials were collapsed, the vocal condition resulted in greater brain activity in the primary auditory cortex, ventrolateral parts of the primary motor cortex, and dorsal parts of cerebellar lobules V/VIIIa/VIIb, all of which are implicated in vocalization (arrowheads labeled “vocalization” in Supplementary Fig. 13a, b ). On the other hand, the manual condition led to greater activity in the dorsomedial parts of the primary motor cortex and somatosensory cortex, and the ventral parts of cerebellar lobules V/VI/VIIIa/VIIIb, all of which which are implicated in right-hand movement (arrowheads labeled “vocalization” in Supplementary Fig. 13a, b bottom ).
Next, to test whether response modality affected the interference effect, we compared the interference effect (incongruent vs. congruent) between the vocal and manual conditions (Fig. 5 ). In the Stroop task, greater brain activity was observed in the primary auditory cortex in the vocal condition than in the manual condition (Fig. 5a and Supplementary Table 3 ). On the other hand, relative to the vocal condition, the manual condition showed greater activity in the primary motor cortex and in cerebellar lobules V/VI, which are implicated in right hand movement. As in the Stroop task, the Swimmy task resulted in greater activity in the primary motor cortex and cerebellar lobules V/VI in the manual condition than in the vocal condition (Fig. 5b and Supplementary Table 4 ). These results suggest that the interference-related activity in the lPFC, PPC, OTC, and cerebellum (crus I/lobule VI and crus II/lobule VIIb) is unaffected by response modalities in both the Stroop and Swimmy tasks.
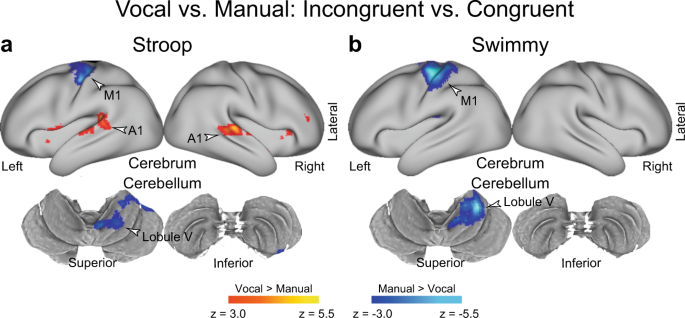
a , b Response type affects the involvement of sensory-motor regions, but not the fronto-cerebellar loop associated with interference resolution. Statistical maps showing a differential interference effect (incongruent vs. congruent) between the vocal and manual conditions. Stroop task ( a ); Swimmy task ( b ). Hot and cool colors indicate greater interference effect in the vocal and manual conditions, respectively. c The Stroop effect predominantly involves the left lPFC and right cerebellum. Statistical maps showing a differential interference effect (incongruent vs. congruent) between the Stroop and Swimmy tasks. Hot and cool colors indicate a greater interference effect in the Stroop and Swimmy tasks, respectively. Formats are similar to those in Fig. 3a .
Differential cerebro-cerebellar involvement in the Stroop and Swimmy tasks
The observations thus far demonstrated following: (1) the interference effects were independent of response modality in both the Stroop and Swimmy tasks; (2) the Stroop and reverse-Stroop effects involved similar cross-hemispheric, lateralized, cerebro-cerebellar mechanisms; and (3) the interference effects in the outline and individual tasks in the Swimmy task involved similar bilateral cerebro-cerebellar mechanisms. To directly compare the interference effects between the Stroop task (Fig. 3a ) and the Swimmy tasks (Fig. 4a ), we contrasted the activation maps between the two tasks. As shown in Fig. 6a and Supplementary Table 5 , in the Stroop task, the interference effect was greater in the lPFC and PPC in the left hemisphere and in the crus II in the right cerebellum. On the other hand, the Swimmy task showed a greater interference effect in the PPC in the right hemisphere. These results provide statistical evidence that while the Stroop effect involves left lateral cortical and right cerebellar regions, the Swimmy effect involves bilateral cortical and cerebellar regions.
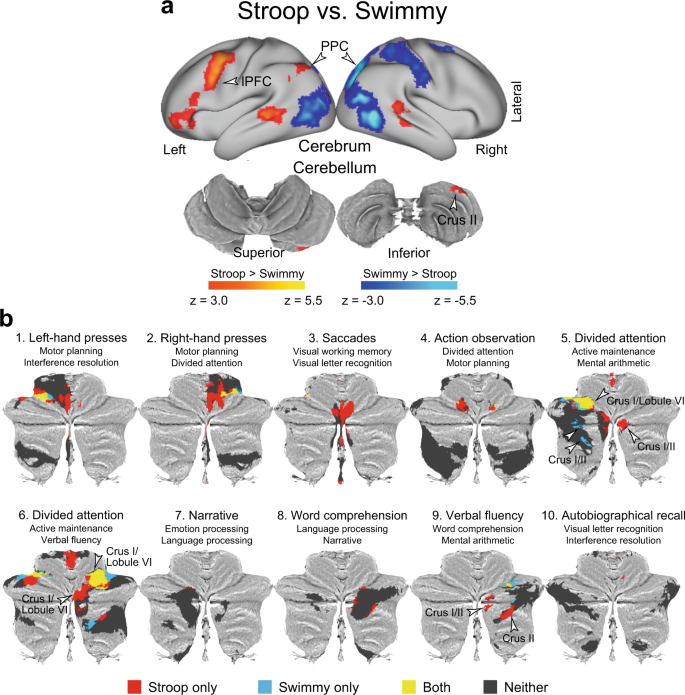
a The Stroop effect predominantly involves the left lPFC and right cerebellum. Statistical maps showing a differential interference effect (incongruent vs. congruent) between the Stroop and Swimmy tasks. Hot and cool colors indicate a greater interference effect in the Stroop and Swimmy tasks, respectively. Formats are similar to those in Fig. 3a . b The current results for the contrast of the incongruent vs. congruent trials in the Stroop and Swimmy tasks ( P < 0.05, FWE-corrected across the whole cerebellum based on non-parametric permutation tests) are for the cerebellar ROIs defined by functional parcellation in a previous study mapped onto 2D flat maps of the cerebellum. The functional labels and IDs of the ROIs above the maps were derived in the previous study. Red: Stroop only; blue: Swimmy only; yellow: both Stroop and Swimmy; gray: neither Stroop nor Swimmy.
The cerebellar involvement in the Stroop effect is associated with language and attentional functions
To functionally characterize the cerebellar involvement, we performed cerebellum-specific imaging analysis (see Methods). An exploratory analysis within the cerebellar atlas space 77 was first performed to identify cerebellar regions involved in the Stroop and Swimmy effects. Then, cerebellar regions were classified into those showing activity (1) only in the Stroop task, (2) only in the Swimmy task, (3) in both tasks, or (4) in neither task. The classified regions were further mapped into ROIs defined by functional parcellation based on task-fMRI data of multi-domain task battery 49 .
As shown in Fig. 6b , crus I and II of Region 9 49 showed significant activity only in the Stroop task. Interestingly, this ROI was previously labeled “verbal fluency” 49 , a language-related function, and was restricted to the right hemisphere. Notably, this region also showed greater activity in the Stroop task than in the Swimmy task in the whole-brain analysis (Fig. 6a ).
In Regions 5 and 6, both of which were labeled “divided attention” 49 , bilateral regions in crus I and lobule VI were active in both tasks. In these ROIs, the Stroop task involved the right hemisphere (crus I and lobule VI), whereas the Swimmy task involved both hemispheres (crus I and lobule VI).
These conjunctions and disjunctions within the ROIs reflect well the statistical z-maps for the contrasts of both the incongruent vs. congruent trials (Supplementary Fig. 14 ) and the Stroop vs. Swimmy tasks (Supplementary Fig. 15 ). Collectively, the results suggest that cerebellar involvement is associated with language-related functionality in the Stroop task and cognitive functionality in both the Stroop and Swimmy tasks.
Statistical z-maps of the cerebellum were also created for the contrast of the incongruent vs. congruent trials in the vocal condition relative to the manual condition, corresponding to the whole-brain analysis in Fig. 5 . Supplementary Fig. 16 shows that Region 2, which was previously labeled “right-hand presses” 49 , demonstrated prominent activity in the manual condition, confirming the whole-brain analysis.
Cross-hemispheric cerebro-cerebellar mechanisms are absent in a large-scale meta-analysis of the Stroop effect
Previous neuroimaging studies of the Stroop effect have reported the involvement of the mPFC/ACC, lPFC, PPC, and OTC 12 , 16 , 23 , 25 , 27 , 56 , 57 , 58 , 59 , 60 , 61 , 62 , 63 , 67 , 78 . To evaluate the current observations in reference to previous studies, we compared our results with those of a large-scale meta-analysis of the Stroop effect 62 . As shown in Fig. 7a (top), the meta-analysis revealed broad regions of involvement in the mPFC/ACC, lPFC, PPC, and OTC. In the current study, on the other hand, the involvement was left-lateralized, but overlapped well with that in the meta-analysis. Additionally, our study showed cerebellar involvement that was absent in the meta-analysis. However, the left lateralization in the cortical regions was less pronounced in the Swimmy task (Fig. 7a bottom) than in the Stroop task. These comparisons suggest that in our study, the results of the Swimmy task, and not those of the Stroop task, are compatible with the findings of the meta-analysis of the Stroop effect.
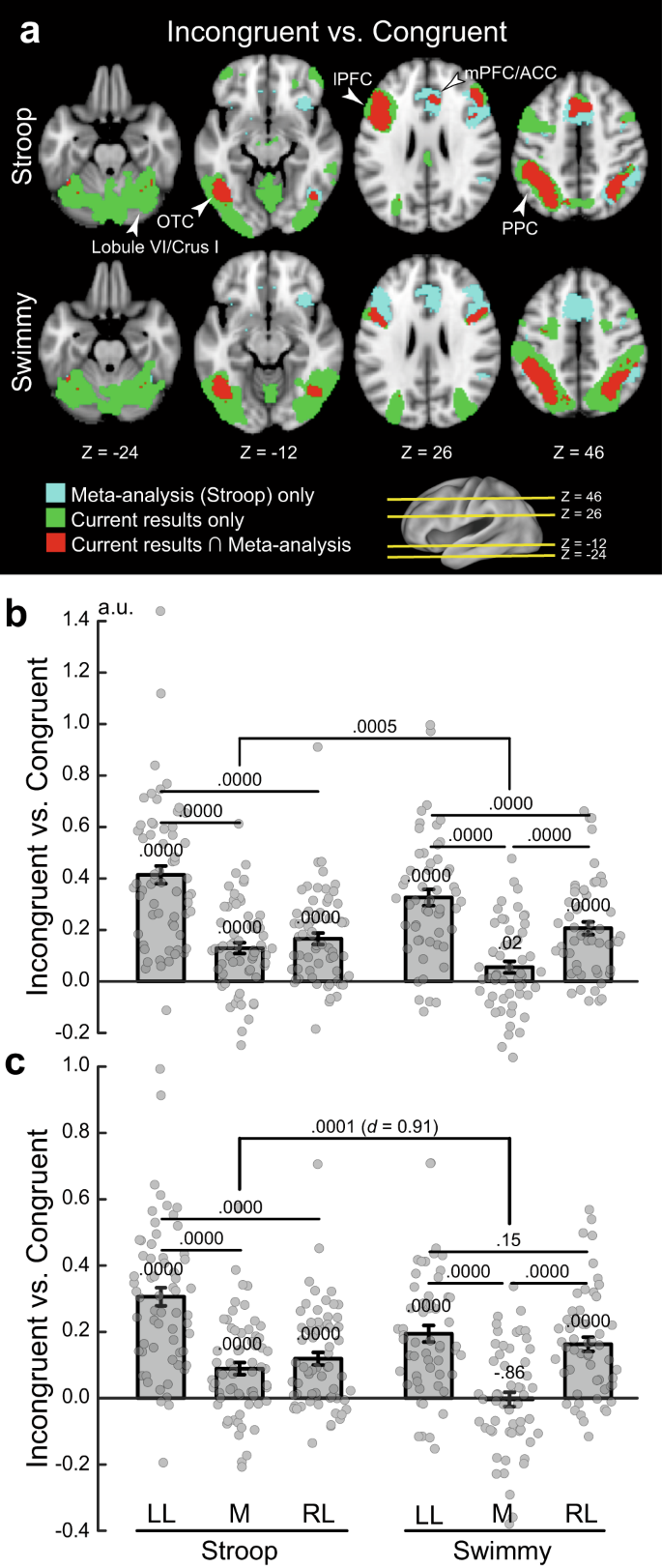
a Meta-analysis maps of the Stroop effect and the current activation maps of interference effects (incongruent vs. congruent) are overlaid on the transverse sections of structural images. Stroop task (top); Swimmy task (bottom). Cyan: meta-analysis maps only; green: current maps only; red: both maps overlapping. The levels of sections are indicated by Z levels at the bottom and yellow lines on the 3D surface of the brain at the right bottom. Arrowheads indicate anatomical locations. b ROI analysis (Stroop: N = 63; Swimmy: N = 55; independent participants). ROIs were defined as left lateral (LL), medial (M), and right lateral (RL) regions based on X axis levels in the meta-analysis maps of the Stroop effect. Stroop task (left); Swimmy task (right). Horizontal and vertical axes indicate ROIs and brain activity, respectively. Rectangular and error bars indicate means and standard errors of the mean across participants, respectively, with individual data overlaid on each rectangular bar. Statistical p values are shown on the top ( t tests, two-tailed, uncorrected). c ROI analysis in which ROIs were defined based on the meta-analysis maps of cognitive control. Statistical procedures and formats are similar to those in b . The sample size is identical to that in b .
To quantitatively evaluate the lateralization, we performed ROI analysis. ROIs were defined as left lateral, medial, and right lateral based on X axis coordinates on the meta-analysis map (see Methods). Then, for each ROI, activity magnitudes for the incongruent vs. congruent trials were extracted for the Stroop and Swimmy tasks. A repeated measures ANOVA with task (Stroop and Swimmy) and region laterality (left and right) revealed a significant main effect of laterality [F(1, 116) = 105.6, P < 0.001; Fig. 7b ], indicating a greater interference effect in the left hemisphere in both the Stroop and Swimmy tasks. The main effect of tasks was not significant [F(1, 116) = 0.42, P = 0.52], suggesting that task demands were comparable in the Stroop and Swimmy tasks. Most importantly, the interaction effect of task and laterality was significant [F(1, 116) = 12.9, P < 0.001], indicating that left lateralization is greater in the Stroop task than in the Swimmy task. These results were consistently observed in the vocal and manual conditions (Supplementary Fig. 17 ).
Hemispheric laterality in cognitive control regions
It is well known that the resolution of cognitive interference requires cognitive control involving the lPFC and PPC 13 , 16 . We thus performed a similar ROI analysis using a meta-analysis map of cognitive control 62 . Again, the current Stroop effects overlapped well with the meta-analysis map in the left hemisphere, whereas the Swimmy effects overlapped well with the meta-analysis map in both hemispheres (Supplementary Fig. 18a ). In the Stroop task, both left and right lateral regions showed significant activity [left: t (62) = 11.1, P < 0.001; right: t (62) = 6.3, P < 0.001], but the activity was greater in the left hemisphere than in the right [ t (62) = 8.4, P < 0.001; Fig. 7c ]. In the Swimmy task, both hemispheres showed significant activation [left: t (54) = 7.8, P < 0.001; right: t (54) = 7.6, P < 0.001], and the activity did not differ between hemispheres [left vs. right: t (54) = 1.4, P = 0.15]. The hemispheric difference in lPFC activity was greater in the Stroop task than in the Swimmy task [ t (116) = 4.9, P < 0.001], with a relatively large effect size (Cohen’s d = 0.91). Post hoc statistical power estimation revealed that the power was high (0.94, alpha rate: 0.001). Because the hemispheric laterality effect in the lPFC was controlled in terms of stimulus verbality (Stroop vs. Swimmy) and laterality (left vs. right), this large effect size and statistical power suggest that greater left lPFC and neocortical activity during the incongruent trials in the Stroop task reflects language functions.
The hemispheric laterality pattern was observed in both the vocal and manual condition. Specifically, the interference effect was greater in the left lateral regions [left vs. right: vocal: t (31) = 4.5, P < 0.001; manual: t (30) = 4.9, P < 0.001], and in the Swimmy task, the interference effect was observed bilaterally, and the activity did not differ between the left and right hemispheres [left vs. right: vocal: t (26) = 1.2, P = 0.22; manual: t (27) = 0.74, P = 0.47] (Supplementary Fig. 18b ). On the other hand, compared to the lateral regions, the interference effect was weaker in the medial regions [Stroop: left lateral vs. medial: vocal: t (31) = 6.1, P < 0.001; manual: t (31) = 5.5, P < 0.001; Swimmy: left lateral vs. medial: vocal: t (26) = 5.4, P < 0.001; manual: t (27) = 3.4, P < 0.01; right lateral vs. medial: vocal: t (26) = 4.2, P < 0.001; manual: t (27) = 3.5, P < 0.01]. Taken together, these results clearly demonstrate that the Stroop effect is associated with the left lateral cortical regions, while bilateral cortical regions are involved in the Swimmy effect, in which stimulus perception does not involve verbal information.
To examine the interference effect in neocortical regions more specifically, the ROIs were divided into lPFC, PPC, OTC, and mPFC/ACC regions (Supplementary Fig. 19 ). In the Stroop task, the interference effect was greater in the left hemisphere than the right hemisphere throughout the lPFC, PPC, and OTC [lPFC: t (62) = 6.8, P < 0.001; PPC: t (62) = 7.9, P < 0.001; OTC: t (62) = 6.8, P < 0.001]. In the Swimmy task, on the other hand, the interference effect in the left and right hemispheres did not differ for either the lPFC or PPC [lPFC: t (54) = 0.66, P = 0.51; PPC: t (54) = 1.34, P = 0.19], whereas in the OTC, the interference effect was greater in the left hemisphere [ t (54) = 4.8, P < 0.001]. The hemispheric difference in lPFC activity was greater in the Stroop task than in the Swimmy task [ t (116) = 4.5, P < 0.001] with a relatively large effect size (Cohen’s d = 0.84).
These results suggest that left lateralization of cortical involvement in the Stroop effect, but not the Swimmy effect (Fig. 7c , Supplementary Fig. 18b ), is derived from activity in the fronto-parietal regions.
This study used multiband imaging that is prone to head motion–induced artifacts, with greater banding with higher multiband factors. To minimize these artifacts, we conducted image processing using ICA-based denoising 79 and motion censoring 80 , 81 , and then re-performed the imaging and connectivity analyses. As shown in Supplementary Figs. 18 – 20 , the results are very similar to those in the original analysis (Figs. 3 – 5 and 6a ), and the original findings were preserved.
The current study examined the neural mechanisms underlying the Stroop effect by manipulating the verbality of the stimulus to be perceived and the response to be made. The resolution of Stroop interference involved the left lateral prefrontal cortex and right cerebellum, but the lateralized involvement was not observed in the non-verbal control task (Fig. 8a ). Resolution of the interference by fronto-cerebellar processes involved excitatory signaling from the prefrontal to cerebellar regions and inhibitory signaling from the cerebellar to prefrontal regions (Fig. 8b ). These findings were unrelated to the verbality of the response generation. Our results suggest that Stroop interference occurs during the perception of language and color information, and is resolved by the coordinated fronto-cerebellar loop that may regulate goal-relevant information (Fig. 8c ).
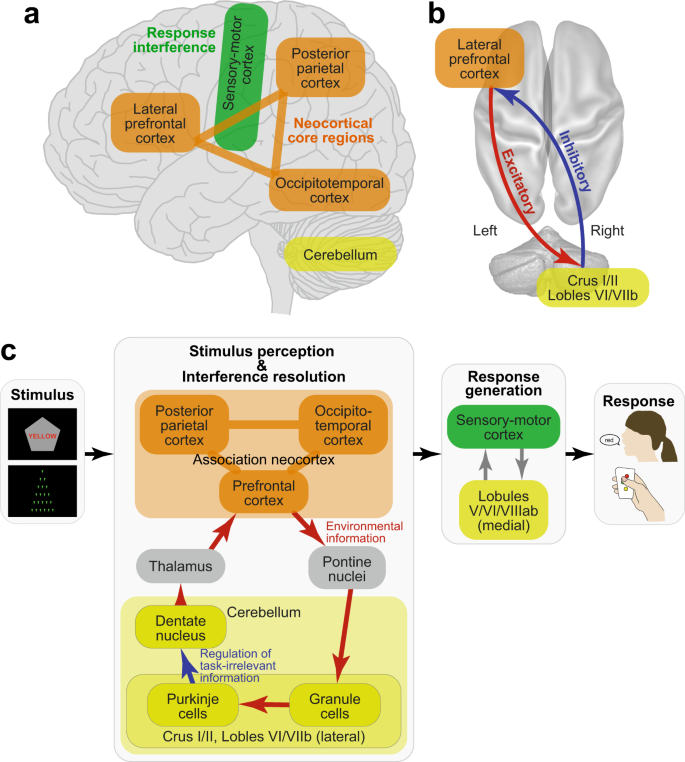
Schematic illustrations of cerebro-cerebellar involvement ( a ) and fronto-cerebellar connectivity ( b ) in the Stroop effect as suggested by the current results. c Schematic processing diagrams of the Stroop/Swimmy effects from stimulus presentation to response execution. Anatomical structure and functionalities of the front-cerebellar loop are speculated based on previous anatomical and neurophysiological evidence.
The neocortical and cerebellar regions are thought to constitute functional-anatomical loops, specifically involving the cerebellar and pontine nuclei and basal ganglia 41 , 44 , 51 . Anatomically, the loop topology has been identified not only between sensorimotor neocortical and ventromedial cerebellar regions, but also between neocortical association regions and dorsolateral cerebellar hemispheres 41 , 44 (Fig. 8c ). A transneuronal tracer study in monkeys identified a loop between the lateral prefrontal cortex and dorsal regions in the lateral cerebellar hemispheres, namely, the crus I/II and lobule VIIb 82 . Interestingly, in our study, homologous regions in the human prefrontal cortex and cerebellum were found to be activated during the incongruent trials (Fig. 3a ), suggesting that a fronto-cerebellar loop is involved in the resolution of Stroop interference (Fig. 8 ).
In relation to task behavior in humans, cortico-cerebellar functional networks have been examined in sensorimotor regions 41 , 50 , 51 , 77 , 83 . Distinct cortical and cerebellar involvement in voluntary movements is reflected in topographical parcellation of resting-state functional connectivity between the motor cortex and cerebellum 50 . Notably, the lateral prefrontal and dorsolateral cerebellar regions involved in the Stroop interference in the current study also showed strong resting-state connectivity (region 12 in ref. 50 ).
A classical PET study revealed that language function is associated with the left lateral frontal cortex and right cerebellum, demonstrating a contra-lateral cerebro-cerebellar involvement 41 , 52 . Neuropsychological studies also showed that damage to the right cerebellum impaired language-related functions 45 , 46 , 84 , 85 . Consistent with these reports, our study demonstrated that a Stroop task involving language functions also relied on cross-hemispheric, lateralized, fronto-cerebellar mechanisms. The functional characterization based on the cerebellum-dedicated imaging analysis (Fig. 6b ) suggests that the Stroop effect involves language and attentional functions implemented in the lateral and dorsal parts of the cerebellar cortex.
One interesting finding regarding the directional functional connectivity observed in this study is that the cerebellum sent inhibitory signals to the prefrontal cortex, whereas the prefrontal cortex sent excitatory signals to the cerebellum, a pattern that was enhanced in the incongruent trials (Figs. 3 c and 8b ). In the cortico-cerebellar loop, Purkinje cells receive excitatory projections from granule cells within the cerebellar cortex, and send inhibitory projections to the deep cerebellar nuclei, which send signals to the thalamus toward neocortical regions 41 , 44 , 51 (Fig. 8c ). Importantly, the inhibitory projections of the Purkinje cells constitute only one output from the cerebellar cortex. Thus, the inhibitory signals from the cerebellum to the prefrontal cortex observed in our study may reflect the functionality of the Purkinje cells within the cerebro-cerebellar loop (Fig. 8c ), although strong fMRI signals in the cerebellum do not reflect Purkinje cell activity alone, and may reflect other neuronal activity of granule cells 41 , 86 . As such, the strong inhibitory signaling from the cerebellum to the prefrontal cortex may help to filter out task-irrelevant signals derived from visual information that interfered with appropriate task performance in the incongruent trials (Fig. 8c ).
Prior neuroimaging studies of the Stroop effect used mainly manual responses and reported involvement of the bilateral and medial prefrontal regions 12 , 24 , 25 , 26 , 63 , 67 , 78 . In particular, statistically significant activations were reported in right hemisphere regions in some studies 87 , 88 , 89 , but other studies identified activations in left hemisphere regions 14 , 21 , 22 , 24 , 26 , 28 or in bilateral regions 25 , 27 , 29 , 90 , 91 . A meta-analysis map of the Stroop effect was created based on relevant studies including those studies 62 . The above findings are inconsistent with the left-lateralized activity in cortical regions in this study, which was found in an exploratory analysis without a priori hypothesis.
Arguments of laterality based only on significant hemisphere-specific activity are not strong because active regions can vary depending on the statistical threshold used. To circumvent this issue, this study directly contrasted activity between the right and left hemispheres, and explored brain regions in which there was greater activity in one hemisphere than in the other (Supplementary Fig. 4 ), as in our previous study 68 .
Importantly, this discrepancy in the Stroop task was observed not only between the meta-analysis and the current vocal condition, but also between the meta-analysis and the current manual condition. Indeed, our results showed that the left-lateralized involvement of association cortices was independent of response modality (Figs. 5 and 8 ). In this regard, the current study may highlight a limitation of the meta-analysis approach, specifically that a collection of indecisive results can yield an unclear conclusion, providing a reasonable lesson that meta-analyses should be performed based on decisive studies.
We acknowledge that our contrast of the incongruent vs. congruent trials could involve a facilitation effect (faster response in the congruent trials than in the neutral trials). However, facilitation is not a concomitant of interference 11 , the facilitation effect is usually weaker than the interference effect 92 , and the interference and facilitation have a common locus 93 . Interestingly, a theoretical study suggested that a low proactive control could lead to reverse facilitation (i.e., faster responses to neutral stimuli than to congruent stimuli) 94 . Thus, we do not think that the facilitation is dominant against the interference in this study.
Previous neuroimaging studies have often used comparisons between the incongruent and congruent trials to minimize oddball effects and to match visual stimuli across conditions 21 , 24 , 27 , 29 , 88 . Interestingly, two studies compared activity between incongruent trials and neutral trials and between congruent trials and neutral trials, and found that prefrontal activity was higher in the incongruent trials than in both the neutral and congruent trials 16 , 26 , which is consistent with the current study.
A neuropsychological study found that damage to the left lPFC impaired performance in the Stroop task 95 , which is consistent with our results. The study also found that damage to the right lPFC impaired performance in a neuropsychological test involving language processing and response inhibition, which agrees with previous studies of response inhibition 96 , 97 .
To characterize and highlight the Stroop effect involving language processing, we developed the Swimmy task as a control task in which the stimulus does not involve language information, like typical flanker tasks 31 , but rather behavioral procedures corresponded to those in the current Stroop task. Whereas the Stroop effect showed unilateral neural involvement, the Swimmy effect showed bilateral involvement, which is consistent with prior neuroimaging studies of flanker tasks 32 , 33 , 36 , 37 , 38 and the Simon tasks 91 , 98 . One of these studies directly compared activity during the Stroop task and the Simon task and found greater activity in the left lPFC during the Stroop task 91 . Our study extends this finding by showing that the right cerebellum is also involved specifically in the Stoop task.
Our results also demonstrated that the involvement was independent of response modality. Collectively, these findings indicate that lateralization is dependent on stimulus modality but not on response modality (Fig. 8 ). Thus, our results suggest that left-lateralized cortical involvement is attributable to the visual word stimulus used in the Stroop task, which is consistent with classical knowledge about language processing in the human brain 17 , 18 . Additionally, right-lateralized cerebellar involvement in the Stroop effect may reflect perceptual language processing, again consistent with prior neuroimaging and neuropsychological studies 41 , 45 , 52 .
Samples were independent across the four conditions (vocal Stroop, manual Stroop, vocal Swimmy, and manual Swimmy), and the size of each sample group was comparable to those in standard fMRI studies. All four groups showed robust effects (Figs. 2 and 5 and Supplementary Figs. 2 , 5 – 10 , 11 , and 14 – 16 ). The current results could contain group-specific effects due to the nature of between-group comparisons, but given the sample size and robust results of each group, the degree of these group-specific effects should be equivalent to those in standard fMRI studies.
Behaviorally, in both the Stroop and Swimmy tasks, the interference effect was greater in the manual conditions than in the vocal conditions (Fig. 2 ). This pattern is opposite to that observed in previous behavioral studies 15 , 19 , 20 , possibly due to the high baseline cognitive demand that was further enhanced in the manual conditions because stimulus-response relationships were not straightforward.
Neuroimaging analyses revealed that in the manual conditions, the interference effects were stronger in the primary motor and somatosensory cortices and the motor-related cerebellar regions 41 , 50 , 51 in both the Stroop and Swimmy tasks (Figs. 3 and 4 ). In contrast, in the vocal condition, the primary auditory cortex showed stronger interference effects. These results suggest that the differences in the behavioral interference effects between the vocal and manual conditions are reflected in the greater activity in the motor and somatosensory regions. Interestingly, the association neocortices (lPFC, PPC, OTC) did not show such differential involvement between response modalities, suggesting that these regions contribute to the resolution of cognitive-language interference in both the vocal and manual conditions (Fig. 8 ). The response modality-dependent interference effects of behavior are attributable to the involvement of the motor and sensory cortices, but not the core mechanisms of interference resolution in the association cortices. Taken together, our imaging results demonstrate that response modality is not a central issue in examining the Stroop effect, as also predicted by classical behavioral studies 11 .
Classical behavioral studies have demonstrated an asymmetric interference pattern in reaction times between the color task (Stroop effect) and the word task (reverse Stroop effect); reaction time prolongation is greater in the Stroop effect than in the reverse Stroop effect (see ref. 11 for a review). In the current vocal Stroop condition, we observed an asymmetric pattern in accuracy but not in reaction times, which is likely due to a speed-accuracy tradeoff. Nonetheless, we did not observe such asymmetry in the manual Stoop task, and observed a strong reverse Stroop effect in both the vocal and manual conditions. Because participants perceived the presented colored word and made a judgment about it, the strong reverse interference effect (Fig. 2 ) may reflect language functions, namely visual word perception and semantic processing.
In the current task, participants first judged the direction of the vertex of a pentagon, and then performed the color task or the word task depending on the vertex direction. This entailed high baseline cognitive demand. Importantly, because the incongruent and congruent trials and the color and word tasks were pseudorandomized in the tasks, the baseline cognitive demand was canceled out when comparing behavior and neuroimaging data between trials and tasks.
Standard Stroop tasks in behavioral and neuroimaging studies have used a blocked design in which participants perform one task (color naming or word reading) continuously. In the block design, because participants are always sure about the upcoming task, they are able to actively maintain task goals (i.e., naming the color by ignoring its word in the color task or reading the word by ignoring its color in the word task), which is referred to as proactive control 99 , 100 . Importantly, previous studies have demonstrated that enhanced proactive control reduces the interference effect in the Stoop task, both behaviorally 101 , 102 , and neurally 29 . Given this evidence, we used an event-related fMRI design in which trial conditions were intermixed in one task block, and matched the visual stimulus, trial frequency, and baseline cognitive demand across the tasks.
In our task design, participants were unsure before each trial about the task to be performed. As a result, proactive control was unavailable, and only reactive control was possible, with the task goal being reactivated in each trial 99 , 100 . In this reactive control situation, because processing of word and color is initiated after the presentation of a trial stimulus, the trial imposes high cognitive control.
The longer reaction times in this study were attributable to the reactive control situation. This situation could produce increased processing of the irrelevant dimension and/or reduce the inhibition of the irrelevant information. Thus, the reactive control situation in our task could have yielded a robust reverse Stroop effect in the current study.
Although our behavioral results were not fully consistent with those of classical behavioral studies regarding Stroop effect asymmetry, a greater Stroop effect in the manual condition, and longer reaction times, we note that our neuroimaging results did not reflect these behavioral patterns (Figs. 3 and 5 – 7 ). We also note that a previous neuroimaging study 67 reported significant differences in both reaction times and accuracy between the Stroop and reverse Stroop effects, and an asymmetric pattern in accuracy, which is consistent with the current study behaviorally.
The resolution of the Stroop effect refers to the critical operation necessary to achieve a behavioral goal in incongruent trials 99 . The resolution is involved in cognitive processing that is theoretically distinct from interference. Specifically, interference refers to a situation where the behavioral goal is provided and the visual stimulus is perceived, but the critical processing to achieve the goal is not yet complete. This distinction entails that interference (Stroop effect) occurs before its resolution. We acknowledge that the limited temporal resolution of fMRI and our task design did not allow us to differentiate these processes. However, this was not the intent of our study (see also Figs. 1 g and 8c ).
A key question in this study is how the Stroop effect occurs and is resolved in the brain. This question concerns not only neural correlates (i.e., where in the brain), but also task-related signal processing (i.e., what signals are in the brain). For the former, we performed exploratory analysis across the brain and identified multiple brain regions, including the lPFC and cerebellum. For the latter, we performed directional functional connectivity analysis based on DCM and found inhibitory–excitatory signaling between the lPFC and cerebellum. As such, this study provided neurophysiological evidence to answer the question.
Participants
Written informed consent was obtained from 119 healthy young participants. Each individual participated in one of the four conditions (vocal Stroop: N = 33, age range: 18–24, eight females; manual Stroop: N = 31, age range 18–23, 11 females; vocal Swimmy: N = 27, age range: 18–23, seven females; manual Swimmy: N = 28, age range: 18–23, nine females). Experimental procedures were approved by the institutional review board of Keio University and Kochi University of Technology. Participants received 2000 yen for participation. All participants were right-handed, had normal color vision, and spoke Japanese as a native language, and had no history of neurological or psychiatric disorders. The study sample size was determined prior to data collection based on pilot experiments and previous relevant studies. One participant in the vocal Stroop experiments was excluded from analyses due to poor behavioral performance (i.e., low accuracy in the incongruent trials; <0.60).
Outline of experimental design
A set of participants performed a Stroop task while undergoing functional MRI (Fig. 1c ). Another set of participants performed the Swimmy task, an interference task in which the stimulus did not involve verbal information (Fig. 1d ). The Swimmy task served as a control condition for the Stroop task. Except for the visual stimulus, identical task procedures were used in the Stroop and Swimmy tasks (Fig. 1e ). During the tasks, participants responded verbally or manually (Fig. 1f ). Thus, the experiments consisted of two stimulus types (Stroop, Swimmy) and two response types (vocal, manual), entailing a 2 × 2 factorial design (Fig. 1g ).
We used a between-subject design rather than a within-subject design to minimize the training effect on task performance, since this effect may change the degree of interference in the Stroop and Swimmy tasks 1 , 11 . In particular, the amount of training for the incongruent trials was equivalent across the four conditions.
Behavioral procedures
In the Stroop task, a colored word and a gray pentagon were simultaneously presented on the screen in each trial (Fig. 1c ). The word, which was composed of Japanese syllabary characters ( kana ), was placed at the center of the pentagon. The colored word and the word color were blue, red, yellow, or green. Participants were required to judge the color or word in each colored word stimulus, depending on which direction in which the pentagon vertex faced. For example, if the vertex pointed upward, participants had to specify the color in the stimulus (color task), and if the vertex pointed downward, they had to specify the word in the stimulus (word task). The color and word in the stimulus were either congruent (color and word were matched) or incongruent (color and word were unmatched). The relationship between the vertex direction and task was counterbalanced across participants.
In contrast to the Stroop task, the stimulus set in the Swimmy task did not involve verbal information. In each trial, a set of small isosceles triangles were presented (Fig. 1d ). The orientation of the vertex with the smallest angle was identical for all triangles. The arrangement of the small, individual triangles formed the outline of a larger, homothetic, isosceles triangle. The outline and individual triangles pointed up, right, down, or left. Participants were required to judge the orientation of the vertex of the individual triangles or that of the large triangle outline, depending on the color of the triangles. For example, if the color of the individual triangles was orange, they had to judge the orientation of the large triangle outline, and if the color of the individual triangles was green, they had to judge the orientation of these triangles. The orientation of the outline triangle was either incongruent or congruent with those of the individual triangles. The relationship between the task and the color of the individual triangles was counterbalanced across participants. This task was referred to as the Swimmy task based on an old picture book in which a number of small fish formed the pattern of a large fish 55 .
Both the Stroop and Swimmy tasks consisted of two tasks (Stroop: color and word; Swimmy: outline and individual) and two congruency levels (incongruent and congruent), entailing a 2 × 2 factorial design. The four conditions were presented pseudorandomly for 2 s followed by a 2-s fixation period (Fig. 1e ). The pseudorandomized design aimed to (1) reduce the proactive control strategy 99 that was proven to reduce behavioral and neural effects in the Stroop task 29 , 101 , 102 , (2) minimize the oddball effect involving the lateral prefrontal cortex 29 , and (3) match visual stimuli and baseline cognitive demands across the tasks.
Participants were instructed to respond as accurately and quickly as possible. Each run consisted of 48 trials, with 12 trials for each of the four conditions. All visual stimuli were presented by E-Prime (Psychology Software Tools, Sharpsburg PA, USA; ver. 2.0.10.356).
Except for the visual stimulus, presentation procedures were identical between the Stroop and Swimmy tasks (Fig. 1e ). Participants responded either vocally or manually (Fig. 1f ). In the vocal conditions, responses were recorded using an MRI-compatible microphone (FORMRI-III, Optoacoustics, Israel). In the manual conditions, participants responded by pressing a button with their right thumb.
Previous studies of the Stroop effect have often used neutral trials (e.g., a colored circle in the color task and an achromatic word in the word task) rather than congruent trials as a control of the incongruent trials. However, it is well known that perception of visual stimuli involves distinct occipitotemporal regions depending on the modality of visual stimulus 103 , 104 , 105 . Additionally, changes in color or shape were found to drive attentional shifts, which involved the lateral prefrontal cortex 16 , 106 . Thus, we designed the Stroop and Swimmy tasks such that visual stimuli were identical across conditions (Fig. 1c, d ), which enabled strict cross-condition comparisons in neuroimaging analysis.
In both tasks, we also equalized the trial frequency in each condition. This frequency control is important to minimize contamination by the oddball effect, which involves the lateral prefrontal cortex 107 . Indeed, previous neuroimaging studies of the Stroop effect showed that a lower frequency of the incongruent trials enhanced activity in broad brain regions, including the lPFC, PPC, and OTC 29 , 108 , and that this activity might be attributable to the oddball effect. Thus, a neutral trial was not optimal to server as a control condition in order to minimize the oddball effect in terms of color, word, and shape.
Training procedure
In all four experiments (stimulus: Stroop or Swimmy; response: vocal or manual), participants practiced by performing 96 trials that were presented in two separate runs (48 trials per run). Thus, the practice conditions for the congruent and incongruent trials were matched across the four experiments.
For the manual Stroop task only, because stimulus-response relations were not straightforward (e.g., red–left, blue–right, green–up, yellow–down; Fig. 1 bottom ), participants received training prior to the task practice in which they were presented with a colored circle patch or a black word, and pressed a corresponding button. This pre-practice training did not involve congruent or incongruent stimuli. In training for the color task, a colored circle patch was presented on a pentagon, and participants pressed a button corresponding to the color. In training for the word task, a color word in black font was presented on a pentagon, and participants were required to press the button corresponding to the color word. Stimulus presentation procedures were identical to those in the Stroop task. The vertex of the pentagon was randomly oriented downward or upward, but did not indicate the task to be performed. Participants performed five runs of the training task. In the other three tasks (i.e., vocal Stroop, manual Swimmy, vocal Swimmy), training for the stimulus-response relationships was unnecessary because the relationships were straightforward. It is important that the training for the stimulus-response relationships in the manual Stroop task did not involve Stroop effects, to avoid contaminating the practice for the congruent and incongruent trials.
Voice recording and response analysis procedures
Vocal responses were recorded through an MRI-compatible noise-reduction microphone. For each trial, recording was started from the trial onset and stopped at the trial offset. The recording sample rate was 22 kHz. Correct and incorrect responses were sorted manually based on the record data, and trials with completely correct vocal responses within the 2-sec response window were classified as correct trials.
Because automatic MRI noise reduction was insufficient to allow for detection of the onset of vocal responses, an off-line band-path filter was applied to reduce scanner-derived noise (Supplementary Fig. 1 ). Then, the vocal onset was defined as the first time point the magnitude of the filtered vocal data exceeded a certain threshold, and the reaction time was defined as the latency from the start of the recording to the vocal onset. The bandwidth of the filter and the threshold were determined manually for each participant by adjustment based on visual inspection of the data and detected vocal onsets.
Behavioral analysis
For each of the Stroop and Swimmy tasks, accuracy and reaction times were calculated for each task (color or word; outline or individual), congruency condition (incongruent or congruent), and response modality (vocal or manual). They were then compared across tasks, congruency conditions, and response modalities within the Stroop or Swimmy task. Statistical tests were performed using SPSS Statistics 25 (IBM Inc. Armonk, NY USA).
Participants completed five runs, each of which consisted of 48 trials (12 trials × 4 conditions). In the vocal Stroop task, participants gave correct responses for 56.5 ± 3.1 (mean ± SD) incongruent color trials, 59.8 ± 0.4 congruent color trials, 58.0 ± 2.0 incongruent word trials, and 59.7 ± 0.5 congruent word trials. In the manual Stroop task, they gave correct responses for 53.3 ± 6.9 incongruent color trials, 58.9 ± 1.8 congruent color trials, 51.7 ± 6.2 incongruent word trials, and 58.7 ± 1.6 congruent word trials. In the vocal Swimmy task, they gave correct responses for 57.8 ± 2.7 incongruent outline trials, 59.6 ± 0.8 congruent outline trials, 58.7 ± 1.5 incongruent individual trials, and 59.5 ± 0.7 congruent individual trials. In the manual Swimmy task, they gave correct responses for 57.4 ± 2.1 incongruent outline trials, 59.9 ± 0.3 congruent outline trials, 59.0 ± 1.2 incongruent individual trials, and 59.7 ± 0.7 congruent individual trials.
The numbers of correct trials provided sufficient power for the current imaging analyses, and were comparable not only to the numbers in neuroimaging studies of the Stroop effect 21 , 22 , 23 , 25 , 26 , 27 , 28 , 29 , but also similar to or greater than those in our previous studies involving univariate activation and DCM analyses during cognitive control tasks 68 , 97 , 109 .
Imaging procedure
MRI scanning was performed using a 3-T MRI scanner (Siemens Prisma, Germany) with a 64-channel head coil. Functional images were acquired using a multiband acceleration echo-planar imaging sequence [repetition time (TR): 743 msec; echo time (TE): 35.6 msec; flip angle (FA): 48 deg; 72 slices; slice thickness: 2 mm; in-plane resolution: 2 × 2 mm; multiband factor: 8]. One functional run lasted 192 s with 277 volume acquisitions. The initial 10 volumes were discarded for analysis to take into account the equilibrium of longitudinal magnetization. High-resolution anatomical images were acquired using an MP-RAGE T1-weighted sequence [TR: 1900 msec; TE = 2.52 msec; FA: 9 deg; 176 slices; slice thickness: 1 mm; in-plane resolution: 1 × 1 mm 2 ].
Image preprocessing
MRI data were analyzed using SPM12 software ( http://fil.ion.ac.uk/spm/ ; ver. 6685) running on Matlab 2017a (Mathworks, Inc. Natick, MA USA). All functional images were first temporally aligned across volumes and runs, and then the anatomical image was coregistered to a mean image of the functional images. The functional images were spatially normalized to a standard MNI template with normalization parameters estimated from the anatomical image. The images were then resampled into 2-mm isotropic voxels, and spatially smoothed with a 6-mm full-width at half-maximum (FWHM) Gaussian kernel.
In order to minimize motion-derived artifacts due to vocal responses, functional images were further preprocessed by general linear model (GLM) estimations with motion parameters and MRI signal time courses (cerebrospinal fluid, white matter, and whole-brain), and their derivatives and quadratics as nuisance regressors 110 , 111 , 112 in fsl_regfilt in implemented in the FSL suite ( http://fmrib.ox.ac.uk/fsl/ ; ver. 5.0.9). Then residual of the nuisance GLM was used for standard GLM estimations to extract events-related brain activity described below. The same noise reduction procedure was applied to imaging data from all the experiments, including the manual conditions.
To further reduce motion-derived artifacts, we applied ICA-based denoising, implemented by ICA-AROMA 79 , and motion censoring 80 , 81 . For motion censoring, we first calculated motion magnitudes as framewise displacement (FD) values 80 . FD values were 0.192 ± 0.085 (mean ± SD) in vocal Stroop, 0.011 ± 0.021 in manual Stroop, 0.196 ± 0.070 in vocal Swimmy, and 0.103 ± 0.032 in manual Swimmy. When FD was thresholded by 0.9 81 , 113 , the rates of images that exceeded the threshold were 1.2 ± 3.6% in vocal Stroop, 0.06 ± 0.1% in manual Stroop, 1.0 ± 2.4% in vocal Swimmy, and 0.1 ± 0.4% in manual Stroop. The numbers of participants with images exceeding the threshold by more than 5% were two in the vocal Stroop task, zero in the manual Stroop task, one in the vocal Swimmy task, and zero in the manual Swimmy task. These results clearly indicate greater FD in vocal conditions. However, even in the vocal conditions, the absolute magnitudes were small compared to those in prior studies 80 , 81 . We next applied motion censoring (scrubbing) based on GLM analysis 113 , 114 . We created a volume-wise regressor encoding 1 for volumes exceeding FD threshold (0.9) and 0 for others. Then, this effect was regressed out using fsl_regfilt .
In this study, because field maps and blip-up/down data were unavailable, we did not perform susceptibility distortion correction. Thus, we note that the accuracy of the spatial identification of the functional locus could be limited, even though we used a modern, standard fMRI scanner with low distortion.
First-level GLM
Because each participant performed one of the four experimental conditions (vocal Stroop, manual Stroop, vocal Swimmy, or manual Swimmy), the four conditions were separately subjected to first-level GLM analyses. The effects of interest were task dimension (Stroop: color and word; Swimmy: outline and individual) and congruency level (incongruent and congruent). The four trial events (2 tasks and 2 congruency levels) with correct responses were coded separately in a GLM. Error trials were also separately coded in the GLM as a nuisance effect. These trial events were time-locked to the onset of visual stimuli and then convolved with the canonical hemodynamic response function (HRF) implemented in SPM. Then, parameters were estimated for each voxel across the whole brain.
Group-level statistics
Maps of parameter estimates were first contrasted within individual participants. The contrast maps were collected from all participants, and were subjected to group-level one- or two-sample t tests based on permutation methods (5000 permutations) implemented in randomize in the FSL suite. Then voxel clusters were identified using a voxel-wise uncorrected threshold of P < 0.001, and the identified voxel clusters were tested for significance with a threshold of P < 0.05 corrected by the family-wise error (FWE) rate across the whole brain. This group analysis procedure was validated to appropriately control the false positive rates in a prior study 115 . The peaks of significant clusters were identified and listed in tables. If multiple peaks were identified within 12 mm, the most statistically significant peak was kept. Statistical maps were created using Connectome Workbench ( https://www.humanconnectome.org/software/connectome-workbench ; ver. 1.4.2) and SUIT 77 ( http://www.diedrichsenlab.org/imaging/suit.htm ; ver. 3.4). Note that the cerebellar surface maps of the whole-brain analysis are created in the MNI space.
Activity lateralization analysis
To examine the hemispheric laterality of task-related activity, we explored brain regions showing greater activity than the contra-lateral homologous regions. For each participant, contrast maps (incongruent vs. congruent) were flipped along the X (left-right) axis and were subtracted from the original non-flipped maps on a voxel-by-voxel basis 68 . Then, the group-level statistical significance was tested within the left hemisphere. Note that statistical correction was performed within one hemisphere because the subtracted maps showed sign-flipped symmetry along the X axis (Supplementary Fig. 4 ).
Exploration was restricted to brain regions in both hemispheres that showed the interference effect ( P < 0.05 uncorrected). This masking procedure ensured that positive and negative laterality effects indicated greater interference effects in the left and right hemispheres, respectively. We applied this procedure separately to the Stroop and Swimmy tasks, with vocal and manual conditions collapsed.
Effective connectivity analysis
DCM analysis 72 implemented in SPM12 was performed in order to examine directional functional connectivity associated with the Stroop and Swimmy effects. DCM allows us to explore the effective connectivity among brain regions under the premise that the brain is a deterministic dynamic system that is subject to environmental inputs and that produces outputs based on the space-state model. The model constructs a nonlinear system involving intrinsic connectivity, task-induced connectivity, and extrinsic inputs. Specifically, a model of neural activity was formulated as a linear time-invariant space-state dynamic system,
where x ( t ) denotes the states of neural activity in k brain regions ( k × 1 vector) at time t , u ( t ) denotes inputs to the system from task events at time t (scalar value), A denotes intrinsic connectivity ( k × k matrix), B denotes effective connectivity ( k × k matrix), and C denotes the direct influence of the task variable on neural activity (direct extrinsic input; k × 1 vector). Because the time derivative of neural activity (left side in Eq. 1 ) is modulated by \(\left[A+u(t)B\right]x(t)\) , the directionality of connectivity is reflected in the A and B matrices. More specifically, the rows and columns of the A and B matrices indicate the target and source of the directionality. The A , B , and C matrices involved k 2 , \(k(k-1)\) , and k parameters, respectively (only non-diagonal elements are parameters for matrix B ). Thus, the model involved 2 k 2 parameters in total. The unit of the connectivity is arbitrary. Next, the neural activity x ( t ) in the model (Eq. 1 ) was transformed as
where λ denotes a nonlinear function providing fMRI signals from neural activity.
Then, parameters of the nonlinear system ( A , B , and C ) are estimated based on fMRI time series and task variables/events. The use of a high temporal resolution sequence for functional imaging (TR = 0.743 s) enabled us to collect a large number of scan frames to increase the signal-to-noise ratio of the DCM analysis 68 , 70 , 73 .
For the Stroop task, we defined ROIs in the left lPFC and the right cerebellum (CER; lobules V/VI and crus II/lobule VIIb) that showed (1) strong activity in the incongruent condition than in the congruent condition (Fig. 3a ), and (2) cross-hemispheric lateralization (Fig. 3b ). Specifically, the lPFC ROIs were defined within Brodmann area (BA) 44. BA 44 is known to implements multiple cognitive control functions involved in the multiple demand system 116 and to serve as a core prefrontal region for language functions 117 .
To ensure that the ROIs were defined independently of the tested data, and to avoid circular analysis 118 , the DCM analysis of the vocal Stroop task used the ROIs defined by the manual Stroop task, and vice versa. The exact coordinates in the left lPFC were (−40, 14, 28; z = 4.67) in the vocal condition, and (−44, 18, 28; z = 6.06) in the manual condition. For the right CER ROIs, the exact coordinates were (crus I/lobule VI: 32, −62, −28; z = 4.96) and (crus II/lobule VIIb: 24, −72, −42; z = 4.85) in the vocal condition and (crus I/lobule VI: 30, −66, −30; z = 5.25) and (crus II/lobule VIIb: 26, −74, −46; z = 5.72) in the manual condition.
For the Swimmy task, we defined lPFC and CER ROIs bilaterally, given their bilateral involvement (Fig. 4a, b ). As in the Stroop task, ROIs for the vocal condition were defined based on the manual condition, and vice versa to avoid circular analysis 75 . The exact coordinates were (left: −54, 12, 30; z = 4.00) and (right: 52, 12, 28; z = 2.47) in the vocal condition, and (left: −48, 8, 26; z = 4.21) and (right: 46, 8, 30; z = 3.73) in the manual condition. For the CER ROIs, the exact coordinates were (right crus I/lobule VI: 20, −68, −20; z = 5.31), (left crus I/lobule VI: − 24, −60, −26; z = 4.62), (right crus II/lobule VIIb: 30, −76, −48; z = 4.37), and (left crus II/lobule VIIb: −28, −70, −48; z = 3.53) in the vocal condition; and (right crus I/lobule VI: 16, −70, −24; z = 5.44), (left crus I/lobule VI: − 22, −62, −26; z = 4.78), (right crus II/lobule VIIb: 28, −74, −46; z = 3.95), and (left crus II/lobule VIIb: −28, −70, −46; z = 3.80) in the manual condition.
It should be noted that the all the lPFC ROIs are included in the meta-analysis map of cognitive control in Neurosynth ( https://neurosynth.org/analyses/terms/cognitive%20control/ ; P < 0.01, FDR-corrected, uniformity test, minimum z value: 8.4).
To examine the robustness of the connectivity results against ROI definition in the lPFC, lPFC ROIs were re-defined based on the meta-analysis map of cognitive control in Neurosynth (association test). Specifically, the center coordinates of the ROIs were defined as those showing peak z-values within BA 44 in the lPFC. The exact coordinates were (−46, 18, 30; z = 5.6) and (46, 18, 32; z = 7.6) in the left and right lPFC, respectively.
ROI images in lPFC and CER were created as spheres with 6-mm radii centered at these coordinates.
We then tested whether the lPFC region sent (or received) a task-related signal toward (or from) the CER region of the target during the Stroop and Swimmy tasks. Signal time courses (1335 scanning frames) of the lPFC and CER ROIs and regressors in events of interest were extracted from first-level GLMs. The events of interest were the onsets of incongruent and congruent trials. We first separately examined task-related connectivity during the incongruent and congruent trials (relative to baseline). Then we tested whether the connectivity was modulated by task parameters. Specifically, to test whether the task-related connectivity differed between the incongruent and congruent trials, the two trials were coded as a parametrical contrast effect (incongruent: 1; congruent: −1). Likewise, similar parametric effects were examined to test manipulations of tasks [i.e., color and word tasks in the Stroop task (color: 1; word: −1); outline and individual tasks in the Swimmy task (outline: 1; individual: −1)]. The input matrix was U mean-centered.
For each trial effect, causal models were defined as those that differed in modulatory effects (i.e., matrix B ) among ROIs. We were interested in strengths of effective connectivity between pairs of ROIs (e.g., lPFC and CER), rather than exploration of the model that best fits to the data. Thus, we considered all theoretically possible models. In theory, there are 2 k(k−1) models that consist of k brain regions. Connectivity matrices reflecting (1) first-order connectivity (i.e., matrix A ), (2) effective changes in coupling induced by the inputs (i.e., matrix B ), and (3) extrinsic inputs (i.e., matrix C ) on MRI signals in ROIs were estimated for each of the models based on DCM analysis implemented in SPM12. When task-related contrast was examined, a parametric regressor (incongruent vs. congruent, color vs. word, or outline vs. individual) was used as an extrinsic effect for effective connectivity between ROIs and ROIs inputs.
In order to estimate the strength of effective connectivity, a Bayesian model reduction method 69 was used. This reduction method reduces the number of models based on model evidence (free energy) and the posterior densities of the models. Specifically, only the models with a minimal posterior density greater than zero were included. These models were then inverted to a fully connected model, and supplemented by second-level parametric empirical Bayes (PEB) 69 to apply empirical priors that remove subjects’ variability from each model.
Next, the parameters of these models were estimated based on Bayesian model averaging (BMA) 72 to estimate group-level statistics. Because the current analysis aimed to identify effective connectivity observed as an average across participants, we used a fixed-effect (FFX) estimation assuming that every participant uses the same model. This is in contrast to using a random effect (RFX) estimation assuming different participants use different models, which is often used to test group differences in effective connectivity 71 . The significance of connectivity was then tested by thresholding at a posterior probability at the 95% confidence interval. We used the uncorrected threshold, because the current analysis aimed to test if connectivity between two specific brain regions was enhanced depending on task manipulation and brain activity, not to identify the model of connectivity among multiple brain regions that best fits to the imaging and behavioral data 68 , 97 , 109 .
ROI analysis
In order to examine consistency between the current study and prior neuroimaging studies, ROI analyses were performed. Statistical maps for a meta-analysis of the Stroop effect and cognitive control were obtained from Neurosynth ( https://neurosynth.org/analyses/terms/stroop/ ; https://neurosynth.org/analyses/terms/cognitive control/). The uniformity-test maps were thresholded using a statistical threshold of P < 0.01 (FWE-corrected). Then ROIs were defined as left lateral, medial, and right lateral regions in the thresholded maps based on MNI X axis coordinates. Then, for each ROI, activity magnitudes were extracted for the contrast between incongruent and congruent trials from individual participants. The extracted activity magnitudes were then averaged across participants, and compared among ROIs, response modalities, and tasks.
A supplementary ROI analysis was performed to compare activity magnitudes in the lPFC, PPC, OTC, and mPFC/ACC regions (Supplementary Fig. 14 ). The left lateral, medial, and right lateral ROIs of the meta-analysis maps were further classified into lPFC, PPC, OTC, and mPFC/ACC ROIs based on anatomical locations in the meta-analysis map of cognitive control.
Imaging analysis of the cerebellum
To examine cerebellar involvement in details, we performed imaging analysis dedicated to the cerebellum based on the SUIT space 77 .
Functional images were first realigned and then coregistered to each individual’s high-resolution structural image. The individual’s cerebellum and brain stem were then extracted from the image based on tissue segmentation and tissue probability calculation implemented in SUIT and SPM12. As previously recommended ( https://www.diedrichsenlab.org/imaging/suit_function.htm ), for each participant an author (KJ) performed visual inspection and confirmed that the cerebellum and brain stem were appropriately extracted.
Next, the extracted structural image was registered to the SUIT template using nonlinear deformation based on Dartel implemented in SUIT/SPM12. Then, based on the estimated transformation matrix and deformation field as well as the cerebellar mask, functional images were registered to the SUIT space, and resampled into 1-mm isotropic voxels. The registered functional images were spatially smoothed using 6-mm FWHM Gaussian kernel, and motion-related denoising was performed, as in the whole-brain preprocessing based on the MNI space.
Single-level GLM analysis of the cerebellar images was then performed, as in the whole-brain analysis. Group-level analysis was performed similarly, but the FWE rate was corrected within the cerebellum. Then, the cerebellar regions showing significant activity in the contrasts of the incongruent vs. congruent trials in the Stroop and Swimmy task were identified ( P < 0.05, FWE-corrected). For the regions showing significant activity in the Stroop or Swimmy tasks, we performed a conjunction and disjunction analysis in which the cerebellar regions were classified into those showing activity (1) only in the Stroop task, (2) only in the Swimmy task, or (3) in both tasks, and created conjunction and disjunction images.
To characterize the functionality of the cerebellar regions showing prominent activity, we performed ROI analysis. ROIs were defined by a functional parcellation of the cerebellum based on a multi-domain task battery 49 . Then, the conjunction and disjunction images were masked by each ROI, and then unfolded to 2D flat maps of the cerebellum.
Reporting summary
Further information on research design is available in the Nature Portfolio Reporting Summary linked to this article.
Data availability
The neuroimaging time series and relevant event-onset data generated in this study have been deposited in the Dryad database ( https://doi.org/10.5061/dryad.msbcc2g2p ) 119 . The behavioral and ROI data generated in this study are provided in the Source Data file. The database of Neurosynth 62 is publicly available ( https://neurosynth.org/ ). Source data are provided with this paper.
Code availability
A Matlab code to perform estimations of single-level GLMs is provided in the Dryad database via the Zenodo repository ( https://doi.org/10.5281/zenodo.7329617 ) 119 .
Stroop, J. R. Studies of interference in serial verbal reactions. J. Exp. Psychol. 18 , 643–662 (1935).
Article Google Scholar
Comalli, P. E., Wapner, S. & Werner, H. Interference effects of Stroop color-word test in childhood, adulthood, and aging. J. Genet. Psychol. 100 , 47–53 (1962).
Klein, G. S. Semantic power measured through the interference of words with color-naming. Am. J. Psychol. 77 , 576–588 (1964).
Article CAS Google Scholar
Jensen, A. R. & Rohwer, W. D. The Stroop color-word test: a review. Acta Psychol. (Amst.) 25 , 36–93 (1966).
Cohen, L. et al. The visual word form area: spatial and temporal characterization of an initial stage of reading in normal subjects and posterior split-brain patients. Brain 123 , 291–307 (2000).
Cohen, L. et al. Language-specific tuning of visual cortex? Functional properties of the Visual Word Form Area. Brain 125 , 1054–1069 (2002).
Marslen-Wilson, W. D. & Tyler, L. K. Morphology, language and the brain: the decompositional substrate for language comprehension. Philos. Trans. R. Soc. Lond. B Biol. Sci. 362 , 823–836 (2007).
Pallier, C., Devauchelle, A. D. & Dehaene, S. Cortical representation of the constituent structure of sentences. Proc. Natl Acad. Sci. USA 108 , 2522–2527 (2011).
Article ADS CAS Google Scholar
Friederici, A. D. The cortical language circuit: from auditory perception to sentence comprehension. Trends Cogn. Sci. 16 , 262–268 (2012).
Vagharchakian, L., Dehaene-Lambertz, G., Pallier, C. & Dehaene, S. A temporal bottleneck in the language comprehension network. J. Neurosci. 32 , 9089–9102 (2012).
MacLeod, C. M. Half a century of research on the Stroop effect: an integrative review. Psychol. Bull. 109 , 163–203 (1991).
Bush, G. et al. The counting Stroop: an interference task specialized for functional neuroimaging-validation study with functional MRI. Hum. Brain Mapp. 6 , 270–282 (1998).
Kiyonaga, A. & Egner, T. The working memory stroop effect: when internal representations clash with external stimuli. Psychol. Sci. 25 , 1619–1629 (2014).
Galer, S. et al. Investigating the neural correlates of the Stroop effect with magnetoencephalography. Brain Topogr. 28 , 95–103 (2015).
Kinoshita, S., De Wit, B. & Norris, D. The magic of words reconsidered: investigating the automaticity of reading color-neutral words in the Stroop task. J. Exp. Psychol. Learn Mem. Cogn. 43 , 369–384 (2017).
Carter, C. S., Mintun, M. & Cohen, J. D. Interference and facilitation effects during selective attention: an H215O PET study of Stroop task performance. Neuroimage 2 , 264–272 (1995).
Sakai, K. L., Homae, F. & Hashimoto, R. Sentence processing is uniquely human. Neurosci. Res. 46 , 273–279 (2003).
Petersen, S. E., Fox, P. T., Posner, M. I., Mintun, M. & Raichle, M. E. Positron emission tomographic studies of the cortical anatomy of single-word processing. Nature 331 , 585–589 (1988).
Logan, G. D., Zbrodoff, N. J. & Williamson, J. Strategies in the color-word Stroop task. Bull. Psychonomic Soc. 22 , 135–138 (1984).
Sharma, D. & McKenna, F. P. Differential components of the manual and vocal Stroop tasks. Mem. Cogn. 26 , 1033–1040 (1998).
Freund, M. C., Bugg, J. M. & Braver, T. S. A representational similarity analysis of cognitive control during color-word stroop. J. Neurosci. 41 , 7388–7402 (2021).
CAS Google Scholar
Taylor, S. F., Kornblum, S., Lauber, E. J., Minoshima, S. & Koeppe, R. A. Isolation of specific interference processing in the Stroop task: PET activation studies. Neuroimage 6 , 81–92 (1997).
Barch, D. M. et al. Anterior cingulate cortex and response conflict: effects of response modality and processing domain. Cereb. Cortex 11 , 837–848 (2001).
Pardo, J. V., Pardo, P. J., Janer, K. W. & Raichle, M. E. The anterior cingulate cortex mediates processing selection in the Stroop attentional conflict paradigm. Proc. Natl Acad. Sci. USA 87 , 256–259 (1990).
Banich, M. T. et al. fMri studies of Stroop tasks reveal unique roles of anterior and posterior brain systems in attentional selection. J. Cogn. Neurosci. 12 , 988–1000 (2000).
Zysset, S., Müller, K., Lohmann, G. & von Cramon, D. Y. Color-word matching stroop task: separating interference and response conflict. Neuroimage 13 , 29–36 (2001).
Egner, T. & Hirsch, J. The neural correlates and functional integration of cognitive control in a Stroop task. Neuroimage 24 , 539–547 (2005).
McKeown, M. J. et al. Spatially independent activity patterns in functional MRI data during the stroop color-naming task. Proc. Natl Acad. Sci. USA 95 , 803–810 (1998).
Leung, H. C., Skudlarski, P., Gatenby, J. C., Peterson, B. S. & Gore, J. C. An event-related functional MRI study of the stroop color word interference task. Cereb. Cortex 10 , 552–560 (2000).
Damasio, A. R. & Geschwind, N. The neural basis of language. Annu Rev. Neurosci. 7 , 127–147 (1984).
Eriksen, B. A. & Eriksen, C. W. Effects of noise letters upon the identification of a target letter in a nonsearch task. Percept. Psychophys. 16 , 143–149 (1974).
Bunge, S. A., Hazeltine, E., Scanlon, M. D., Rosen, A. C. & Gabrieli, J. D. Dissociable contributions of prefrontal and parietal cortices to response selection. Neuroimage 17 , 1562–1571 (2002).
Morimoto, H. M. et al. On verbal/nonverbal modality dependence of left and right inferior prefrontal activation during performance of flanker interference task. J. Cogn. Neurosci. 20 , 2006–2014 (2008).
van Veen, V., Cohen, J. D., Botvinick, M. M., Stenger, V. A. & Carter, C. S. Anterior cingulate cortex, conflict monitoring, and levels of processing. Neuroimage 14 , 1302–1308 (2001).
Wei, P., Szameitat, A. J., Müller, H. J., Schubert, T. & Zhou, X. The neural correlates of perceptual load induced attentional selection: an fMRI study. Neuroscience 250 , 372–380 (2013).
Hazeltine, E., Poldrack, R. & Gabrieli, J. D. Neural activation during response competition. J. Cogn. Neurosci. 12 , 118–129 (2000).
Fan, J., Flombaum, J. I., McCandliss, B. D., Thomas, K. M. & Posner, M. I. Cognitive and brain consequences of conflict. Neuroimage 18 , 42–57 (2003).
Wager, T. D. et al. Common and unique components of response inhibition revealed by fMRI. Neuroimage 27 , 323–340 (2005).
Miller, E. K. & Cohen, J. D. An integrative theory of prefrontal cortex function. Annu Rev. Neurosci. 24 , 167–202 (2001).
Daniels, C., Witt, K., Wolff, S., Jansen, O. & Deuschl, G. Rate dependency of the human cortical network subserving executive functions during generation of random number series–a functional magnetic resonance imaging study. Neurosci. Lett. 345 , 25–28 (2003).
Diedrichsen, J., King, M., Hernandez-Castillo, C., Sereno, M. & Ivry, R. B. Universal transform or multiple functionality? Understanding the contribution of the human cerebellum across task domains. Neuron 102 , 918–928 (2019).
Liddle, P. F., Kiehl, K. A. & Smith, A. M. Event-related fMRI study of response inhibition. Hum. Brain Mapp. 12 , 100–109 (2001).
Schall, U. et al. Functional brain maps of Tower of London performance: a positron emission tomography and functional magnetic resonance imaging study. Neuroimage 20 , 1154–1161 (2003).
Strick, P. L., Dum, R. P. & Fiez, J. A. Cerebellum and nonmotor function. Annu Rev. Neurosci. 32 , 413–434 (2009).
Kansal, K. et al. Structural cerebellar correlates of cognitive and motor dysfunctions in cerebellar degeneration. Brain 140 , 707–720 (2017).
Google Scholar
Schmahmann, J. D. & Sherman, J. C. The cerebellar cognitive affective syndrome. Brain 121 , 561–579 (1998).
D’Mello, A. M., Gabrieli, J. D. E. & Nee, D. E. Evidence for hierarchical cognitive control in the human cerebellum. Curr. Biol. 30 , 1881–1892.e1883 (2020).
Tedesco, A. M. et al. The cerebellar cognitive profile. Brain 134 , 3672–3686 (2011).
King, M., Hernandez-Castillo, C. R., Poldrack, R. A., Ivry, R. B. & Diedrichsen, J. Functional boundaries in the human cerebellum revealed by a multi-domain task battery. Nat. Neurosci. 22 , 1371–1378 (2019).
Buckner, R. L., Krienen, F. M., Castellanos, A., Diaz, J. C. & Yeo, B. T. The organization of the human cerebellum estimated by intrinsic functional connectivity. J. Neurophysiol. 106 , 2322–2345 (2011).
Buckner, R. L. The cerebellum and cognitive function: 25 years of insight from anatomy and neuroimaging. Neuron 80 , 807–815 (2013).
Petersen, S. E., Fox, P. T., Posner, M. I., Mintun, M. & Raichle, M. E. Positron emission tomographic studies of the processing of singe words. J. Cogn. Neurosci. 1 , 153–170 (1989).
Simon, J. R. Reactions towards the source of stimulation. J. Exp. Psychol. 81 , 174–176 (1969).
Navon, D. Forest before trees: the precedence of global features in visual perception. Cogn. Psychol. 9 , 353–383 (1977).
Article ADS Google Scholar
Lionni, L. Swimmy (Alfred A. Knopf, 1963).
Botvinick, M. M., Braver, T. S., Barch, D. M., Carter, C. S. & Cohen, J. D. Conflict monitoring and cognitive control. Psychol. Rev. 108 , 624–652 (2001).
Carter, C. S. et al. Parsing executive processes: strategic vs. evaluative functions of the anterior cingulate cortex. Proc. Natl Acad. Sci. USA 97 , 1944–1948 (2000).
Bush, G., Luu, P. & Posner, M. I. Cognitive and emotional influences in anterior cingulate cortex. Trends Cogn. Sci. 4 , 215–222 (2000).
MacDonald, A. W., Cohen, J. D., Stenger, V. A. & Carter, C. S. Dissociating the role of the dorsolateral prefrontal and anterior cingulate cortex in cognitive control. Science 288 , 1835–1838 (2000).
MacLeod, C. M. & MacDonald, P. A. Interdimensional interference in the Stroop effect: uncovering the cognitive and neural anatomy of attention. Trends Cogn. Sci. 4 , 383–391 (2000).
Milham, M. P. et al. The relative involvement of anterior cingulate and prefrontal cortex in attentional control depends on nature of conflict. Brain Res. Cogn. Brain Res. 12 , 467–473 (2001).
Yarkoni, T., Poldrack, R. A., Nichols, T. E., Van Essen, D. C. & Wager, T. D. Large-scale automated synthesis of human functional neuroimaging data. Nat. Methods 8 , 665–670 (2011).
Zoccatelli, G., Beltramello, A., Alessandrini, F., Pizzini, F. B. & Tassinari, G. Word and position interference in stroop tasks: a behavioral and fMRI study. Exp. Brain Res. 207 , 139–147 (2010).
Dunbar, K. & MacLeod, C. M. A horse race of a different color: Stroop interference patterns with transformed words. J. Exp. Psychol. Hum. Percept. Perform. 10 , 622–639 (1984).
Fennell, A. & Ratcliff, R. Does response modality influence conflict? Modelling vocal and manual response Stroop interference. J. Exp. Psychol. Learn Mem. Cogn. 45 , 2098–2119 (2019).
Glaser, M. O. & Glaser, W. R. Time course analysis of the Stroop phenomenon. J. Exp. Psychol. Hum. Percept. Perform. 8 , 875–894 (1982).
Song, Y. & Hakoda, Y. An fMRI study of the functional mechanisms of Stroop/reverse-Stroop effects. Behav. Brain Res. 290 , 187–196 (2015).
Tsumura, K., Aoki, R., Takeda, M., Nakahara, K. & Jimura, K. Cross-hemispheric complementary prefrontal mechanisms during task switching under perceptual uncertainty. J. Neurosci. 41 , 2197–2213 (2021).
Friston, K. J. et al. Bayesian model reduction and empirical Bayes for group (DCM) studies. Neuroimage 128 , 413–431 (2016).
Penny, W. D., Stephan, K. E., Mechelli, A. & Friston, K. J. Comparing dynamic causal models. Neuroimage 22 , 1157–1172 (2004).
Penny, W. D. et al. Comparing families of dynamic causal models. PLoS Comput Biol. 6 , e1000709 (2010).
Article MathSciNet Google Scholar
Friston, K. J., Harrison, L. & Penny, W. Dynamic causal modelling. Neuroimage 19 , 1273–1302 (2003).
Stephan, K. E. et al. Ten simple rules for dynamic causal modeling. Neuroimage 49 , 3099–3109 (2010).
Smith, S. M. et al. Network modelling methods for FMRI. Neuroimage 54 , 875–891 (2011).
Kriegeskorte, N., Simmons, W. K., Bellgowan, P. S. & Baker, C. I. Circular analysis in systems neuroscience: the dangers of double dipping. Nat. Neurosci. 12 , 535–540 (2009).
Fink, G. R. et al. Where in the brain does visual attention select the forest and the trees? Nature 382 , 626–628 (1996).
Diedrichsen, J. & Zotow, E. Surface-based display of volume-averaged cerebellar imaging data. PLoS One 10 , e0133402 (2015).
van Veen, V. & Carter, C. S. Separating semantic conflict and response conflict in the Stroop task: a functional MRI study. Neuroimage 27 , 497–504 (2005).
Pruim, R. H. R. et al. ICA-AROMA: a robust ICA-based strategy for removing motion artifacts from fMRI data. Neuroimage 112 , 267–277 (2015).
Power, J. D., Barnes, K. A., Snyder, A. Z., Schlaggar, B. L. & Petersen, S. E. Spurious but systematic correlations in functional connectivity MRI networks arise from subject motion. Neuroimage 59 , 2142–2154 (2012).
Siegel, J. S. et al. Statistical improvements in functional magnetic resonance imaging analyses produced by censoring high-motion data points. Hum. Brain Mapp. 35 , 1981–1996 (2014).
Kelly, R. M. & Strick, P. L. Cerebellar loops with motor cortex and prefrontal cortex of a nonhuman primate. J. Neurosci. 23 , 8432–8444 (2003).
Bostan, A. C., Dum, R. P. & Strick, P. L. Cerebellar networks with the cerebral cortex and basal ganglia. Trends Cogn. Sci. 17 , 241–254 (2013).
Fiez, J. A., Petersen, S. E., Cheney, M. K. & Raichle, M. E. Impaired non-motor learning and error detection associated with cerebellar damage. A single case study. Brain 115 , 155–178 (1992).
Gebhart, A. L., Petersen, S. E. & Thach, W. T. Role of the posterolateral cerebellum in language. Ann. N. Y. Acad. Sci. 978 , 318–333 (2002).
Thomsen, K., Piilgaard, H., Gjedde, A., Bonvento, G. & Lauritzen, M. Principal cell spiking, postsynaptic excitation, and oxygen consumption in the rat cerebellar cortex. J. Neurophysiol. 102 , 1503–1512 (2009).
Bench, C. J. et al. Investigations of the functional anatomy of attention using the Stroop test. Neuropsychologia 31 , 907–922 (1993).
Schulte, T. et al. Double dissociation between action-driven and perception-driven conflict resolution invoking anterior versus posterior brain systems. Neuroimage 48 , 381–390 (2009).
Egner, T. Right ventrolateral prefrontal cortex mediates individual differences in conflict-driven cognitive control. J. Cogn. Neurosci. 23 , 3903–3913 (2011).
Spielberg, J. M. et al. Trait approach and avoidance motivation: lateralized neural activity associated with executive function. Neuroimage 54 , 661–670 (2011).
Peterson, B. S. et al. An event-related functional MRI study comparing interference effects in the Simon and Stroop tasks. Brain Res. Cogn. Brain Res. 13 , 427–440 (2002).
Glaser, W. R. & Düngelhoff, F. J. The time course of picture-word interference. J. Exp. Psychol. Hum. Percept. Perform. 10 , 640–654 (1984).
Roelofs, A. Attention and facilitation: converging information versus inadvertent reading in Stroop task performance. J. Exp. Psychol. Learn Mem. Cogn. 36 , 411–422 (2010).
Kalanthroff, E., Davelaar, E. J., Henik, A., Goldfarb, L. & Usher, M. Task conflict and proactive control: a computational theory of the Stroop task. Psychol. Rev. 125 , 59–82 (2018).
Cipolotti, L. et al. Inhibition processes are dissociable and lateralized in human prefrontal cortex. Neuropsychologia 93 , 1–12 (2016).
Aron, A. R., Robbins, T. W. & Poldrack, R. A. Inhibition and the right inferior frontal cortex. Trends Cogn. Sci. 8 , 170–177 (2004).
Tsumura, K. et al. Perceptual uncertainty alternates top-down and bottom-up fronto-temporal network signaling during response inhibition. J. Neurosci. 42 , 4567–4579 (2022).
Cespón, J., Hommel, B., Korsch, M. & Galashan, D. The neurocognitive underpinnings of the Simon effect: an integrative review of current research. Cogn. Affect Behav. Neurosci. 20 , 1133–1172 (2020).
Braver, T. S. The variable nature of cognitive control: a dual mechanisms framework. Trends Cogn. Sci. 16 , 106–113 (2012).
Jimura, K., Locke, H. S. & Braver, T. S. Prefrontal cortex mediation of cognitive enhancement in rewarding motivational contexts. Proc. Natl Acad. Sci. USA 107 , 8871–8876 (2010).
Kalanthroff, E., Avnit, A., Henik, A., Davelaar, E. J. & Usher, M. Stroop proactive control and task conflict are modulated by concurrent working memory load. Psychon. Bull. Rev. 22 , 869–875 (2015).
Spinelli, G. & Lupker, S. J. Proactive control in the Stroop task: a conflict-frequency manipulation free of item-specific, contingency-learning, and color-word correlation confounds. J. Exp. Psychol. Learn Mem. Cogn. 47 , 1550–1562 (2021).
Zeki, S. et al. A direct demonstration of functional specialization in human visual cortex. J. Neurosci. 11 , 641–649 (1991).
Morita, T. et al. The neural substrates of conscious color perception demonstrated using fMRI. Neuroimage 21 , 1665–1673 (2004).
Dehaene, S. & Cohen, L. The unique role of the visual word form area in reading. Trends Cogn. Sci. 15 , 254–262 (2011).
Serences, J. T. & Yantis, S. Spatially selective representations of voluntary and stimulus-driven attentional priority in human occipital, parietal, and frontal cortex. Cereb. Cortex 17 , 284–293 (2007).
Brázdil, M. et al. Combined event-related fMRI and intracerebral ERP study of an auditory oddball task. Neuroimage 26 , 285–293 (2005).
Melcher, T. & Gruber, O. Oddball and incongruity effects during Stroop task performance: a comparative fMRI study on selective attention. Brain Res. 1121 , 136–149 (2006).
Tsumura, K. et al. Reversible fronto-occipitotemporal signaling complements task encoding and switching under ambiguous cues. Cereb. Cortex 32 , 1911–1931 (2022).
Ciric, R. et al. Benchmarking of participant-level confound regression strategies for the control of motion artifact in studies of functional connectivity. Neuroimage 154 , 174–187 (2017).
Keerativittayayut, R., Aoki, R., Sarabi, M.T., Jimura, K. & Nakahara, K. Large-scale network integration in the human brain tracks temporal fluctuations in memory encoding performance. Elife 7 , e32696 (2018).
Tanaka, D. et al. Self-controlled choice arises from dynamic prefrontal signals that enable future anticipation. J. Neurosci. 40 , 9736–9750 (2020).
Davis, T., Goldwater, M. & Giron, J. From concrete examples to abstract relations: the rostrolateral prefrontal cortex integrates novel examples into relational categories. Cereb. Cortex 27 , 2652–2670 (2017).
Bakkour, A., Lewis-Peacock, J. A., Poldrack, R. A. & Schonberg, T. Neural mechanisms of cue-approach training. Neuroimage 151 , 92–104 (2017).
Eklund, A., Nichols, T. E. & Knutsson, H. Cluster failure: why fMRI inferences for spatial extent have inflated false-positive rates. Proc. Natl Acad. Sci. USA 113 , 7900–7905 (2016).
Camilleri, J. A. et al. Definition and characterization of an extended multiple-demand network. Neuroimage 165 , 138–147 (2018).
Sakai, K. L. Language acquisition and brain development. Science 310 , 815–819 (2005).
Zhang, J., Kriegeskorte, N., Carlin, J. D. & Rowe, J. B. Choosing the rules: distinct and overlapping frontoparietal representations of task rules for perceptual decisions. J. Neurosci. 33 , 11852–11862 (2013).
Okayasu, M. et al. The Stroop effect involves an excitatory–inhibitory fronto–cerebellar loop. Dryad, Dataset , https://doi.org/10.5061/dryad.msbcc5062g5062p (2022).
Download references
Acknowledgements
This study was supported by Kakenhi (JPSP: 21H05060, 20K07727, 19H04914, 17K01989, 17H05957, and 17H00891 to KJ; 20H00521, 21K18267 to MT), and a grant from Uehara Memorial Foundation, a grant from Takeda Science Foundation, (PI: Koji Jimura). We thank Ms. Maho Hosono for technical assistance. We also thank Ms. Maoko Yamanaka for administrative assistance.
Author information
Authors and affiliations.
Department of Biosciences and Informatics, Keio University, Yokohama, Japan
Moe Okayasu, Tensei Inukai, Daiki Tanaka, Kaho Tsumura, Reiko Shintaki & Koji Jimura
Research Center for Brain Communication, Kochi University of Technology, Kami, Japan
Masaki Takeda, Kiyoshi Nakahara & Koji Jimura
Department of Informatics, Gunma University, Maebashi, Japan
Koji Jimura
You can also search for this author in PubMed Google Scholar
Contributions
M.O., D.T., and K.J. designed the experiment and study. M.O., D.T., R.S., and K.J. collected the data. M.O., T.I., K.T., and K.J. analyzed the data. M.O., M.T., K.N., and K.J. wrote the manuscript.
Corresponding author
Correspondence to Koji Jimura .
Ethics declarations
Competing interests.
The authors declare no competing interests.
Peer review
Peer review information.
Nature Communications thanks Laurent Grégoire, Shao-Min Hung, and Joseph Orr for their contribution to the peer review of this work. Peer reviewer reports are available.
Additional information
Publisher’s note Springer Nature remains neutral with regard to jurisdictional claims in published maps and institutional affiliations.
Supplementary information
Supplementary information, peer review file, reporting summary, source data, source data, rights and permissions.
Open Access This article is licensed under a Creative Commons Attribution 4.0 International License, which permits use, sharing, adaptation, distribution and reproduction in any medium or format, as long as you give appropriate credit to the original author(s) and the source, provide a link to the Creative Commons license, and indicate if changes were made. The images or other third party material in this article are included in the article’s Creative Commons license, unless indicated otherwise in a credit line to the material. If material is not included in the article’s Creative Commons license and your intended use is not permitted by statutory regulation or exceeds the permitted use, you will need to obtain permission directly from the copyright holder. To view a copy of this license, visit http://creativecommons.org/licenses/by/4.0/ .
Reprints and permissions
About this article
Cite this article.
Okayasu, M., Inukai, T., Tanaka, D. et al. The Stroop effect involves an excitatory–inhibitory fronto-cerebellar loop. Nat Commun 14 , 27 (2023). https://doi.org/10.1038/s41467-022-35397-w
Download citation
Received : 20 February 2022
Accepted : 30 November 2022
Published : 11 January 2023
DOI : https://doi.org/10.1038/s41467-022-35397-w
Share this article
Anyone you share the following link with will be able to read this content:
Sorry, a shareable link is not currently available for this article.
Provided by the Springer Nature SharedIt content-sharing initiative
This article is cited by
Structural brain correlates of sleep microstructure in spinocerebellar ataxia type 2 and its role on clinical phenotype.
- Roberto Rodríguez-Labrada
- Nalia Canales-Ochoa
- Luis Velázquez-Pérez
The Cerebellum (2024)
By submitting a comment you agree to abide by our Terms and Community Guidelines . If you find something abusive or that does not comply with our terms or guidelines please flag it as inappropriate.
Quick links
- Explore articles by subject
- Guide to authors
- Editorial policies
Sign up for the Nature Briefing newsletter — what matters in science, free to your inbox daily.

Stroop Effect
- Reference work entry
- First Online: 01 January 2022
- pp 6748–6755
- Cite this reference work entry
- J. Antonio Salamanca 4 &
- David A. Washburn 3 , 4
71 Accesses
The Stroop task and its corresponding effect are among the best known, most frequently researched phenomena in cognitive psychology (MacLeod 1991 ; Washburn 2016 ). In its most recognizable form, participants are required to indicate, as quickly as possible, the print color of a series of words, some of which are themselves incompatible color words. J. Ridley Stroop ( 1935 ) studied interference in color naming by comparing performance in baseline conditions to a condition in which the color stimuli were themselves incongruent color words (e.g., the word GREEN printed in red, the word BLUE printed in yellow, and so forth, where the corresponding correct response would be “red, yellow, …”). This interference, manifest as slower responding and increased errors, has become known as “the Stroop effect” and was originally interpreted as reflecting the difference in response strength between the conflicting stimulus dimensions. Each word itself has a single, strongly associated...
This is a preview of subscription content, log in via an institution to check access.
Access this chapter
Institutional subscriptions
Beran, M. J., & Heimbauer, L. A. (2015). A longitudinal assessment of vocabulary retention in symbol-competent chimpanzees (Pan troglodytes). PLoS One, 10 (2), e0118408.
Article PubMed PubMed Central Google Scholar
Beran, M. J., Washburn, D. A., & Rumbaugh, D. M. (2007). A Stroop-like effect in color-naming of color-word lexigrams by a chimpanzee (Pan troglodyte). The Journal of General Psychology, 134 (2), 217–228.
Article PubMed Google Scholar
Besner, D., & Coltheart, M. (1979). Ideographic and alphabetic processing in skilled reading of English. Neuropsychologia, 17 (5), 467–472.
Bramlett-Parker, J., & Washburn, D. A. (2016). Can rhesus monkey learn executive attention? Behavioral Sciences, 6 (2), 11.
Article PubMed Central PubMed Google Scholar
Callery, R., Klein, E. D., & Washburn, D. A. (2015). Learning and use of symbols by rhesus monkeys ( Macaca mulatta ): Better than chance but worse than expected. Paper presented at the annual meeting of the Southern Society for Philosophy and Psychology, New Orleans, LA.
Google Scholar
Cole, M. W., Yeung, N., Freiwald, W. A., & Botvinick, M. (2009). Cingulate cortex: Diverging data from humans and monkeys. Trends in Neurosciences, 32 (11), 566–574.
Cavoto, K. K., & Cook, R. G. (2001). Cognitive precedence for local information in hierarchical stimulus processing by pigeons. Journal of Experimental Psychology: Animal Behavior Processes, 27 (1), 3.
PubMed Google Scholar
Cohen, D. J. (2009). Integers do not automatically activate their quantity representation. Psychonomic Bulletin & Review, 16 (2), 332–336.
Article Google Scholar
Fan, J., McCandliss, B. D., Sommer, T., Raz, A., & Posner, M. I. (2002). Testing the efficiency and independence of attentional networks. Journal of Cognitive Neuroscience, 14 (3), 340–347. https://doi.org/10.1162/089892902317361886 .
Hopkins, W. D., & Washburn, D. A. (2002). Matching visual stimuli on the basis of global and local features by chimpanzees (Pan troglodytes) and rhesus monkeys (Macaca mulatta). Animal Cognition, 5 (1), 27–31.
Kane, M. J., & Engle, R. W. (2003). Working-memory capacity and the control of attention: The contributions of goal neglect, response competition, and task set to Stroop interference. Journal of Experimental Psychology: General, 132 (1), 47–70. https://doi.org/10.1037/0096-3445.132.1.47 .
Kaufmann, L., & Nuerk, H. C. (2006). Interference effects in a numerical Stroop paradigm in 9-to 12-year-old children with ADHD-C. Child Neuropsychology, 12 (3), 223–243.
Lauwereyns, J., Koizumi, M., Sakagami, M., Hikosaka, O., Kobayashi, S., & Tsutsui, K. I. (2000). Interference from irrelevant features on visual discrimination by macaques (Macaca fuscata): A behavioral analogue of the human Stroop effect. Journal of Experimental Psychology: Animal Behavior Processes, 26 (3), 352.
MacLeod, C. M. (1991). Half a century of research on the Stroop effect: An integrative review. Psychological Bulletin, 109 (2), 163–203. https://doi.org/10.1037/0033-2909.109.2.163 .
MacLeod, C. M. (1992). The Stroop task: The “gold standard” of attentional measures. Journal of Experimental Psychology: General, 121 (1), 12–14.
Mansouri, F. A., Tanaka, K., & Buckley, M. J. (2009). Conflict-induced behavioural adjustment: A clue to the executive functions of the prefrontal cortex. Nature Reviews Neuroscience, 10 (2), 141–152.
Michelet, T., Bioulac, B., Guehl, D., Escola, L., & Burbaud, P. (2007). Impact of commitment on performance evaluation in the rostral cingulate motor area. Journal of Neuroscience, 27 (28), 7482–7489.
Michelet, T., Bioulac, B., Guehl, D., Goillandeau, M., & Burbaud, P. (2009). Single medial prefrontal neurons cope with error. PLoS One, 4 (7), e6240.
Michelet, T., Bioulac, B., Langbour, N., Goillandeau, M., Guehl, D., & Burbaud, P. (2015). Electrophysiological correlates of a versatile executive control system in the monkey anterior cingulate cortex. Cerebral Cortex, 26 (4), 1684–1697.
Pardo, J. V., Pardo, P. J., Janer, K. W., & Raichle, M. E. (1990). The anterior cingulate cortex mediates processing selection in the Stroop attentional conflict paradigm. Proceedings of the National Academy of Sciences, 87 (1), 256–259.
Posner, M. I., & Raichle, M. E. (1994). Images of mind . New York: Scientific American Library/Scientific American Books.
Posner, M. I., Rothbart, M. K., Sheese, B. E., & Tang, Y. (2007). The anterior cingulate gyrus and the mechanism of self-regulation. Cognitive, Affective, & Behavioral Neuroscience, 7 (4), 391–395.
Ray, B. A. (1969). Selective attention: The effects of combining stimuli which control incompatible behavior. Journal of the Experimental Analysis of Behavior, 12 (4), 539–550.
Rosas, J. M., Matías Gámez, A., León, S. P., Tirado, G. G., & Byron Nelson, J. (2017). Of rats and people: A select comparative analysis of cue competition, the contents of learning, and retrieval. International Journal of Psychology & Psychological Therapy, 17 (2), 223–244.
Rumbaugh, D. M. (Ed.). (1977). Language learning by a chimpanzee: The Lana project . Academic, New York.
Rumbaugh, D. M., & Washburn, D. A. (2003). Intelligence of apes and other rational beings . Yale University Press, New Haven, CT.
Simon, J. R., & Berbaum, K. (1990). Effect of conflicting cues on information processing: The ‘Stroop effect’ vs. the ‘Simon effect’. Acta Psychologica, 73 (2), 159–170.
Stroop, J. R. (1935). Studies of interference in serial verbal reactions. Journal of Experimental Psychology, 18 (6), 643–662. https://doi.org/10.1037/h0054651 .
Washburn, D. A. (1994). Stroop-like effects for monkeys and humans: Processing speed or strength of association? Psychological Science, 5 (6), 375–379. https://doi.org/10.1111/j.1467-9280.1994.tb00288.x .
Washburn, D. A. (2016). The Stroop effect at 80: The competition between stimulus control and cognitive control. Journal of the Experimental Analysis of Behavior, 105 (1), 3–13. https://doi.org/10.1002/jeab.194 .
Washburn, D. A., & Rumbaugh, D. M. (1991). Ordinal judgments of numerical symbols by macaques (Macaca mulatta). Psychological Science, 2 (3), 190–193. https://doi.org/10.1111/j.1467-9280.1991.tb00130.x .
Washburn, D. A., & Taglialatela, L. A. (2012). The competition for attention in humans and other animals. In T. Zentall & E. A. Wasserman (Eds.), The Oxford handbook of comparative cognition (pp. 100–116). New York: Oxford University Press.
Wolach, A. H., McHale, M. A., & Tarlea, A. (2004). Numerical Stroop effect. Perceptual and Motor Skills, 98 (1), 67–77.
Zentall, T. R. (2005). Selective and divided attention in animals. Behavioural Processes, 69 (1), 1–15.
Download references
Author information
Authors and affiliations.
Covenant College, Lookout Mountain, GA, USA
David A. Washburn
Georgia State University, Atlanta, GA, USA
J. Antonio Salamanca & David A. Washburn
You can also search for this author in PubMed Google Scholar

Corresponding author
Correspondence to David A. Washburn .
Editor information
Editors and affiliations.
Department of Psychology, Oakland University, Rochester, MI, USA
Jennifer Vonk
Todd K. Shackelford
Section Editor information
University of Manitoba, Winnipeg, MB, Canada
Dawson Clary
Rights and permissions
Reprints and permissions
Copyright information
© 2022 Springer Nature Switzerland AG
About this entry
Cite this entry.
Salamanca, J.A., Washburn, D.A. (2022). Stroop Effect. In: Vonk, J., Shackelford, T.K. (eds) Encyclopedia of Animal Cognition and Behavior. Springer, Cham. https://doi.org/10.1007/978-3-319-55065-7_1480
Download citation
DOI : https://doi.org/10.1007/978-3-319-55065-7_1480
Published : 20 May 2022
Publisher Name : Springer, Cham
Print ISBN : 978-3-319-55064-0
Online ISBN : 978-3-319-55065-7
eBook Packages : Behavioral Science and Psychology Reference Module Humanities and Social Sciences Reference Module Business, Economics and Social Sciences
Share this entry
Anyone you share the following link with will be able to read this content:
Sorry, a shareable link is not currently available for this article.
Provided by the Springer Nature SharedIt content-sharing initiative
- Publish with us
Policies and ethics
- Find a journal
- Track your research
REVIEW article
The stroop color and word test.
- 1 “Rita Levi Montalcini” Department of Neuroscience, University of Turin, Turin, Italy
- 2 IRCCS Istituto Auxologico Italiano, Ospedale San Giuseppe, Piancavallo, Italy
- 3 CiMeC Center for the Mind/Brain Sciences, University of Trento, Rovereto, Italy
The Stroop Color and Word Test (SCWT) is a neuropsychological test extensively used to assess the ability to inhibit cognitive interference that occurs when the processing of a specific stimulus feature impedes the simultaneous processing of a second stimulus attribute, well-known as the Stroop Effect. The aim of the present work is to verify the theoretical adequacy of the various scoring methods used to measure the Stroop effect. We present a systematic review of studies that have provided normative data for the SCWT. We referred to both electronic databases (i.e., PubMed, Scopus, Google Scholar) and citations. Our findings show that while several scoring methods have been reported in literature, none of the reviewed methods enables us to fully assess the Stroop effect. Furthermore, we discuss several normative scoring methods from the Italian panorama as reported in literature. We claim for an alternative scoring method which takes into consideration both speed and accuracy of the response. Finally, we underline the importance of assessing the performance in all Stroop Test conditions (word reading, color naming, named color-word).
Introduction
The Stroop Color and Word Test (SCWT) is a neuropsychological test extensively used for both experimental and clinical purposes. It assesses the ability to inhibit cognitive interference, which occurs when the processing of a stimulus feature affects the simultaneous processing of another attribute of the same stimulus ( Stroop, 1935 ). In the most common version of the SCWT, which was originally proposed by Stroop in the 1935, subjects are required to read three different tables as fast as possible. Two of them represent the “congruous condition” in which participants are required to read names of colors (henceforth referred to as color-words) printed in black ink (W) and name different color patches (C). Conversely, in the third table, named color-word (CW) condition, color-words are printed in an inconsistent color ink (for instance the word “red” is printed in green ink). Thus, in this incongruent condition, participants are required to name the color of the ink instead of reading the word. In other words, the participants are required to perform a less automated task (i.e., naming ink color) while inhibiting the interference arising from a more automated task (i.e., reading the word; MacLeod and Dunbar, 1988 ; Ivnik et al., 1996 ). This difficulty in inhibiting the more automated process is called the Stroop effect ( Stroop, 1935 ). While the SCWT is widely used to measure the ability to inhibit cognitive interference; previous literature also reports its application to measure other cognitive functions such as attention, processing speed, cognitive flexibility ( Jensen and Rohwer, 1966 ), and working memory ( Kane and Engle, 2003 ). Thus, it may be possible to use the SCWT to measure multiple cognitive functions.
In the present article, we present a systematic review of the SCWT literature in order to assess the theoretical adequacy of the different scoring methods proposed to measure the Stroop effect ( Stroop, 1935 ). We focus on Italian literature, which reports the use of several versions of the SCWT that vary in in terms of stimuli, administration protocol, and scoring methods. Finally, we attempt to indicate a score method that allows measuring the ability to inhibit cognitive interference in reference to the subjects' performance in SCWT.
We looked for normative studies of the SCWT. All studies included a healthy adult population. Since our aim was to understand the various available scoring methods, no studies were excluded on the basis of age, gender, and/or education of participants, or the specific version of SCWT used (e.g., short or long, computerized or paper). Studies were identified using electronic databases and citations from a selection of relevant articles. The electronic databases searched included PubMed (All years), Scopus (All years) and Google Scholar (All years). The last search was run on the 22nd February, 2017, using the following search terms: “Stroop; test; normative.” All studies written in English and Italian were included.
Two independent reviewers screened the papers according to their titles and abstracts; no disagreements about suitability of the studies was recorded. Thereafter, a summary chart was prepared to highlight mandatory information that had to be extracted from each report (see Table 1 ).
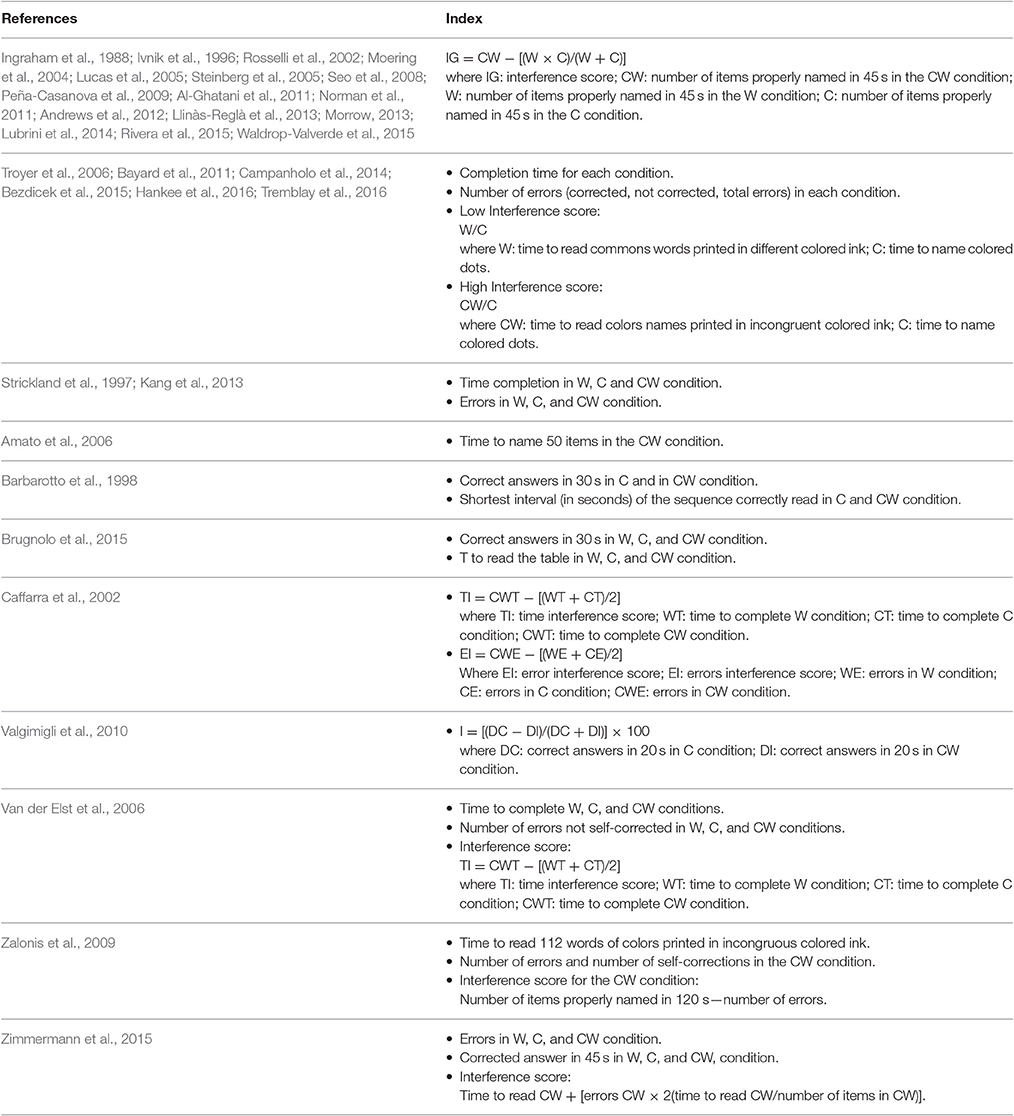
Table 1. Summary of data extracted from reviewed articles; those related to the Italian normative data are in bold .
One Author extracted data from papers while the second author provided further supervision. No disagreements about extracted data emerged. We did not seek additional information from the original reports, except for Caffarra et al. (2002) , whose full text was not available: relevant information have been extracted from Barletta-Rodolfi et al. (2011) .
We extracted the following information from each article:
• Year of publication.
• Indexes whose normative data were provided.
Eventually, as regards the variables of interest, we focused on those scores used in the reviewed studies to assess the performance at the SCWT.
We identified 44 articles from our electronic search and screening process. Eleven of them were judged inadequate for our purpose and excluded. Four papers were excluded as they were written in languages other than English or Italian ( Bast-Pettersen, 2006 ; Duncan, 2006 ; Lopez et al., 2013 ; Rognoni et al., 2013 ); two were excluded as they included children ( Oliveira et al., 2016 ) and a clinical population ( Venneri et al., 1992 ). Lastly, we excluded six Stroop Test manuals, since not entirely procurable ( Trenerry et al., 1989 ; Artiola and Fortuny, 1999 ; Delis et al., 2001 ; Golden and Freshwater, 2002 ; Mitrushina et al., 2005 ; Strauss et al., 2006a ). At the end of the selection process we had 32 articles suitable for review (Figure 1 ).
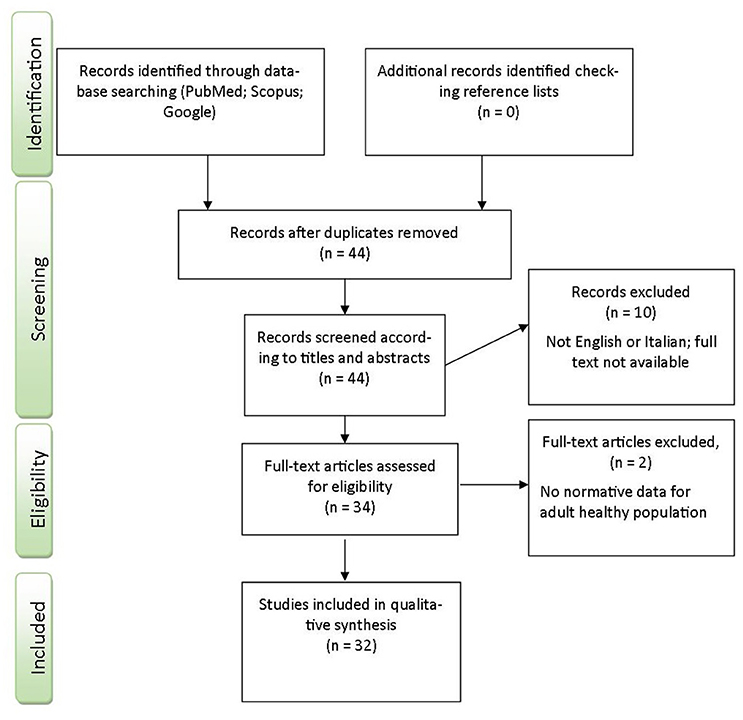
Figure 1. Flow diagram of studies selection process .
From the systematic review, we extracted five studies with Italian normative data. Details are reported in Table 1 . Of the remaining 27 studies that provide normative data for non-Italian populations, 16 studies ( Ivnik et al., 1996 ; Ingraham et al., 1988 ; Rosselli et al., 2002 ; Moering et al., 2004 ; Lucas et al., 2005 ; Steinberg et al., 2005 ; Seo et al., 2008 ; Peña-Casanova et al., 2009 ; Al-Ghatani et al., 2011 ; Norman et al., 2011 ; Andrews et al., 2012 ; Llinàs-Reglà et al., 2013 ; Morrow, 2013 ; Lubrini et al., 2014 ; Rivera et al., 2015 ; Waldrop-Valverde et al., 2015 ) adopted the scoring method proposed by Golden (1978) . In this method, the number of items correctly named in 45 s in each conditions is calculated (i.e., W, C, CW). Then the predicted CW score (Pcw) is calculated using the following formula:
equivalent to:
Then, the Pcw value is subtracted from the actual number of items correctly named in the incongruous condition (CW) (i.e., IG = CW − Pcw): this procedure allows to obtain an interference score (IG) based on the performance in both W and C conditions. Thus, a negative IG value represents a pathological ability to inhibit interference, where a lower score means greater difficulty in inhibiting interference.
Six articles ( Troyer et al., 2006 ; Bayard et al., 2011 ; Campanholo et al., 2014 ; Bezdicek et al., 2015 ; Hankee et al., 2016 ; Tremblay et al., 2016 ) adopted the Victoria Stroop Test. In this version, three conditions are assessed: the C and the CW correspond to the equivalent conditions of the original version of the test ( Stroop, 1935 ), while the W condition includes common words which do not refer to colors. This condition represents an intermediate inhibition condition, as the interference effect between the written word and the color name is not present. In this SCWT form ( Strauss et al., 2006b ), for each condition, the completion time and the number of errors (corrected, non-corrected, and total errors) are recorded and two interference scores are computed:
Five studies ( Strickland et al., 1997 ; Van der Elst et al., 2006 ; Zalonis et al., 2009 ; Kang et al., 2013 ; Zimmermann et al., 2015 ) adopted different SCWT versions. Three of them ( Strickland et al., 1997 ; Van der Elst et al., 2006 ; Kang et al., 2013 ) computed, independently, the completion time and the number of errors for each condition. Additionally, Van der Elst et al. (2006) , computed an interference score based on the speed performance only:
where WT, CT, and CWT represent the time to complete the W, C, and CW table, respectively. Zalonis et al. (2009) recorded: (i) the time; (ii) the number of errors and (iii) the number of self-corrections in the CW. Moreover, they computed an interference score subtracting the number of errors in the CW conditions from the number of items properly named in 120 s in the same table. Lastly, Zimmermann et al. (2015) computed the number of errors and the number of correct answers given in 45 s in each conditions. Additionally, they calculated an interference score derived by the original scoring method provided by Stroop (1935) .
Of the five studies ( Barbarotto et al., 1998 ; Caffarra et al., 2002 ; Amato et al., 2006 ; Valgimigli et al., 2010 ; Brugnolo et al., 2015 ) that provide normative data for the Italian population, two are originally written in Italian ( Caffarra et al., 2002 ; Valgimigli et al., 2010 ), while the others are written in English ( Barbarotto et al., 1998 ; Amato et al., 2006 ; Brugnolo et al., 2015 ). An English translation of the title and abstract of Caffarra et al. (2002) is available. Three of the studies consider the performance only on the SCWT ( Caffarra et al., 2002 ; Valgimigli et al., 2010 ; Brugnolo et al., 2015 ) while the others also include other neuropsychological tests in the experimental assessment ( Barbarotto et al., 1998 ; Amato et al., 2006 ). The studies are heterogeneous in that they differ in terms of administered conditions, scoring procedures, number of items, and colors used. Three studies adopted a 100-items version of the SCWT ( Amato et al., 2006 ; Valgimigli et al., 2010 ; Brugnolo et al., 2015 ) which is similar to the original version proposed by Stroop (1935) . In this version, in every condition (i.e., W, C, CW), items are arranged in a matrix of 10 × 10 columns and rows; the colors are red, green, blue, brown, and purple. However, while two of these studies administered the W, C, and CW conditions once ( Amato et al., 2006 ; Valgimigli et al., 2010 ), Barbarotto et al. (1998) administered the CW table twice, requiring participants to read the word during the first administration and then to name the ink color during the consecutive administration. Additionally, they also administered a computerized version of the SCWT in which 40 stimuli are presented in each condition; red, blue, green, and yellow are used. Valgimigli et al. (2010) and Caffarra et al. (2002) administered shorter paper versions of the SCWT including only three colors (i.e., red, blue, green). More specifically, the former administered only the C and CW conditions including 60 items each, arranged in six columns of 10 items. The latter employed a version of 30 items for each condition (i.e., W, C, CW), arranged in three columns of 10 items each.
Only two of the five studies assessed and provided normative data for all the conditions of the SCWT (i.e., W, C, CW; Caffarra et al., 2002 ; Brugnolo et al., 2015 ), while others provide only partial results. Valgimigli et al. (2010) provided normative data only for the C and CW condition, while Amato et al. (2006) and Barbarotto et al. (1998) administered all the SCWT conditions (i.e., W, C, CW) but provide normative data only for the CW condition, and the C and CW condition respectively.
These studies use different methods to compute subjects' performance. Some studies record the time needed, independently in each condition, to read all ( Amato et al., 2006 ) or a fixed number ( Valgimigli et al., 2010 ) of presented stimuli. Others consider the number of correct answers produced in a fixed time (30 s; Amato et al., 2006 ; Brugnolo et al., 2015 ). Caffarra et al. (2002) and Valgimigli et al. (2010) provide a more complex interference index that relates the subject's performance in the incongruous condition with the performance in the others. In Caffarra et al. (2002) , two interference indexes based on reading speed and accuracy, respectively, are computed using the following formula:
Furthermore, in Valgimigli et al. (2010) an interference score is computed using the formula:
where DC represents the correct answers produced in 20 s in naming colors and DI corresponds to the correct answers achieved in 20 s in the interference condition. However, they do not take into account the performance on the word reading condition.
According to the present review, multiple SCWT scoring methods are available in literature, with Golden's (1978) version being the most widely used. In the Italian literature, the heterogeneity in SCWT scoring methods increases dramatically. The parameters of speed and accuracy of the performance, essential for proper detection of the Stroop Effect, are scored differently between studies, thus highlighting methodological inconsistencies. Some of the reviewed studies score solely the speed of the performance ( Amato et al., 2006 ; Valgimigli et al., 2010 ). Others measure both the accuracy and speed of performance ( Barbarotto et al., 1998 ; Brugnolo et al., 2015 ); however, they provide no comparisons between subjects' performance on the different SCWT conditions. On the other hand, Caffarra et al. (2002) compared performance in the W, C, and CW conditions; however, they computed speed and accuracy independently. Only Valgimigli et al. (2010) present a scoring method in which an index merging speed and accuracy is computed for the performance in all the conditions; however, the Authors assessed solely the performance in the C and the CW conditions, neglecting the subject's performance in the W condition.
In our opinion, the reported scoring methods impede an exhaustive description of the performance on the SCWT, as suggested by clinical practice. For instance, if only the reading time is scored, while accuracy is not computed ( Amato et al., 2006 ) or is computed independently ( Caffarra et al., 2002 ), the consequences of possible inhibition difficulties on the processing speed cannot be assessed. Indeed, patients would report a non-pathological reading speed in the incongruous condition, despite extremely poor performance, even if they do not apply the rule “naming ink color,” simply reading the word (e.g., in CW condition, when the stimulus is the word/red/printed in green ink, patient says “Red” instead of “Green”). Such behaviors provide an indication of the failure to maintain consistent activation of the intended response in the incongruent Stroop condition, even if the participants properly understand the task. Such scenarios are often reported in different clinical populations. For example, in the incongruous condition, patients with frontal lesions ( Vendrell et al., 1995 ; Stuss et al., 2001 ; Swick and Jovanovic, 2002 ) as well as patients affected by Parkinson's Disease ( Fera et al., 2007 ; Djamshidian et al., 2011 ) reported significant impairments in terms of accuracy, but not in terms of processing speed. Counting the number of correct answers in a fixed time ( Amato et al., 2006 ; Valgimigli et al., 2010 ; Brugnolo et al., 2015 ) may be a plausible solution.
Moreover, it must be noted that error rate (and not the speed) is an index of inhibitory control ( McDowd et al., 1995 ) or an index of ability to maintain the tasks goal temporarily in a highly retrievable state ( Kane and Engle, 2003 ). Nevertheless, computing exclusively the error rate (i.e., the accuracy in the performance), without measuring the speed of performance, would be insufficient for an extensive evaluation of the performance in the SCWT. In fact, the behavior in the incongruous condition (i.e., CW) may be affected by difficulties that are not directly related to an impaired ability to suppress the interference process, which may lead to misinterpretation of the patient's performance. People affected by color-blindness or dyslexia would represent the extreme case. Nonetheless, and more ordinarily, slowness, due to clinical circumstances like dysarthria, mood disorders such as depression, or collateral medication effect, may irremediably affect the performance in the SCWT. In Parkinson's Disease, ideomotor slowness ( Gardner et al., 1959 ; Jankovic et al., 1990 ) impacts the processing speed in all SCWT conditions, determining a global difficulty in the response execution rather than a specific impairment in the CW condition ( Stacy and Jankovic, 1992 ; Hsieh et al., 2008 ). Consequently, it seems necessary to relate the performance in the incongruous condition to word reading and color naming abilities, when inhibition capability has to be assessed, as proposed by Caffarra et al. (2002) . In this method the W score and C score were subtracted from CW score. However, as previously mentioned, the scoring method suggested by Caffarra et al. (2002) computes errors and speed separately. Thus, so far, none of the proposed Italian normative scoring methods seem adequate to assess patients' performance in the SCWT properly and informatively.
Examples of more suitable interference scores can be found in non-Italian literature. Stroop (1935) proposed that the ability to inhibit cognitive interference can be measured in the SCWT using the formula:
where, total time is the overall time for reading; mean time per word is the overall time for reading divided by the number of items; and the number of uncorrected errors is the number of errors not spontaneously corrected. Gardner et al. (1959) also propose a similar formula:
where 100 refers to the number of stimuli used in this version of the SCWT. When speed and errors are computed together, the correct recognition of patients who show difficulties in inhibiting interference despite a non-pathological reading time, increases. However, both the mentioned scores ( Stroop, 1935 ; Mitrushina et al., 2005 ) may be susceptible to criticism ( Jensen and Rohwer, 1966 ). In fact, even though accuracy and speed are merged into a global score in these studies ( Stroop, 1935 ; Mitrushina et al., 2005 ), they are not computed independently. In Gardner et al. (1959) the number of errors are computed in relation to the mean time per item and then added to the total time, which may be redundant and lead to a miscomputation.
The most adopted scoring method in the international panorama is Golden (1978) . Lansbergen et al. (2007) point out that the index IG might not be adequately corrected for inter-individual differences in the reading ability, despite its effective adjustment for color naming. The Authors highlight that the reading process is more automated in expert readers, and, consequently, they may be more susceptible to interference ( Lansbergen et al., 2007 ), thus, requiring that the score is weighted according to individual reading ability. However, experimental data suggests that the increased reading practice does not affect the susceptibility to interference in SCWT ( Jensen and Rohwer, 1966 ). Chafetz and Matthews (2004) 's article might be useful for a deeper understanding of the relationship between reading words and naming colors, but the debate about the role of reading ability on the inhibition process is still open. The issue about the role of reading ability on the SCWT performance cannot be adequately satisfied even if the Victoria Stroop Test scoring method ( Strauss et al., 2006b ) is adopted, since the absence of the standard W condition.
In the light of the previous considerations, we recommend that a scoring method for the SCWT should fulfill two main requirements. First, both accuracy and speed must be computed for all SCWT conditions. And secondly, a global index must be calculated to relate the performance in the incongruous condition to reading words and color naming abilities. The first requirement can be achieved by counting the number of correct answers in each condition in within a fixed time ( Amato et al., 2006 ; Valgimigli et al., 2010 ; Brugnolo et al., 2015 ). The second requirement can be achieved by subtracting the W score and C score from CW score, as suggested by Caffarra et al. (2002) . None of the studies reviewed satisfies both these requirements.
According to the review, the studies with Italian normative data present different theoretical interpretations of the SCWT scores. Amato et al. (2006) and Caffarra et al. (2002) describe the SCWT score as a measure of the fronto-executive functioning, while others use it as an index of the attentional functioning ( Barbarotto et al., 1998 ; Valgimigli et al., 2010 ) or of general cognitive efficiency ( Brugnolo et al., 2015 ). Slowing to a response conflict would be due to a failure of selective attention or a lack in the cognitive efficiency instead of a failure of response inhibition ( Chafetz and Matthews, 2004 ); however, the performance in the SCWT is not exclusively related to concentration, attention or cognitive effectiveness, but it relies to a more specific executive-frontal domain. Indeed, subjects have to process selectively a specific visual feature blocking out continuously the automatic processing of reading ( Zajano and Gorman, 1986 ; Shum et al., 1990 ), in order to solve correctly the task. The specific involvement of executive processes is supported by clinical data. Patients with anterior frontal lesions, and not with posterior cerebral damages, report significant difficulties in maintaining a consistent activation of the intended response ( Valgimigli et al., 2010 ). Furthermore, Parkinson's Disease patients, characterized by executive dysfunction due to the disruption of dopaminergic pathway ( Fera et al., 2007 ), reported difficulties in SCWT despite unimpaired attentional abilities ( Fera et al., 2007 ; Djamshidian et al., 2011 ).
According to the present review, the heterogeneity in the SCWT scoring methods in international literature, and most dramatically in Italian literature, seems to require an innovative, alternative and unanimous scoring system to achieve a more proper interpretation of the performance in the SCWT. We propose to adopt a scoring method in which (i) the number of correct answers in a fixed time in each SCWT condition (W, C, CW) and (ii) a global index relative to the CW performance minus reading and/or colors naming abilities, are computed. Further studies are required to collect normative data for this scoring method and to study its applicability in clinical settings.
Author Contributions
Conception of the work: FS. Acquisition of data: ST. Analysis and interpretation of data for the work: FS and ST. Writing: ST, and revising the work: FS. Final approval of the version to be published and agreement to be accountable for all aspects of the work: FS and ST.
Conflict of Interest Statement
The authors declare that the research was conducted in the absence of any commercial or financial relationships that could be construed as a potential conflict of interest.
Acknowledgments
The Authors thank Prerana Sabnis for her careful proofreading of the manuscript.
Al-Ghatani, A. M., Obonsawin, M. C., Binshaig, B. A., and Al-Moutaery, K. R. (2011). Saudi normative data for the Wisconsin Card Sorting test, Stroop test, test of non-verbal intelligence-3, picture completion and vocabulary (subtest of the wechsler adult intelligence scale-revised). Neurosciences 16, 29–41.
PubMed Abstract | Google Scholar
Amato, M. P., Portaccio, E., Goretti, B., Zipoli, V., Ricchiuti, L., De Caro, M. F., et al. (2006). The Rao's brief repeatable battery and stroop test: normative values with age, education and gender corrections in an Italian population. Mult. Scler. 12, 787–793. doi: 10.1177/1352458506070933
PubMed Abstract | CrossRef Full Text | Google Scholar
Andrews, K., Shuttleworth-Edwards, A., and Radloff, S. (2012). Normative indications for Xhosa speaking unskilled workers on the Trail Making and Stroop Tests. J. Psychol. Afr. 22, 333–341. doi: 10.1080/14330237.2012.10820538
CrossRef Full Text | Google Scholar
Artiola, L., and Fortuny, L. A. I. (1999). Manual de Normas Y Procedimientos Para la Bateria Neuropsicolog . Tucson, AZ: Taylor & Francis.
Barbarotto, R., Laiacona, M., Frosio, R., Vecchio, M., Farinato, A., and Capitani, E. (1998). A normative study on visual reaction times and two Stroop colour-word tests. Neurol. Sci. 19, 161–170. doi: 10.1007/BF00831566
Barletta-Rodolfi, C., Gasparini, F., and Ghidoni, E. (2011). Kit del Neuropsicologo Italiano . Bologna: Società Italiana di Neuropsicologia.
Bast-Pettersen, R. (2006). The Hugdahl Stroop Test: A normative stud y involving male industrial workers. J. Norwegian Psychol. Assoc. 43, 1023–1028.
Bayard, S., Erkes, J., and Moroni, C. (2011). Collège des psychologues cliniciens spécialisés en neuropsychologie du languedoc roussillon (CPCN Languedoc Roussillon). Victoria Stroop Test: normative data in a sample group of older people and the study of their clinical applications in the assessment of inhibition in Alzheimer's disease. Arch. Clin. Neuropsychol. 26, 653–661. doi: 10.1093/arclin/acr053
Bezdicek, O., Lukavsky, J., Stepankova, H., Nikolai, T., Axelrod, B. N., Michalec, J., et al. (2015). The Prague Stroop Test: normative standards in older Czech adults and discriminative validity for mild cognitive impairment in Parkinson's disease. J. Clin. Exp. Neuropsychol. 37, 794–807. doi: 10.1080/13803395.2015.1057106
Brugnolo, A., De Carli, F., Accardo, J., Amore, M., Bosia, L. E., Bruzzaniti, C., et al. (2015). An updated Italian normative dataset for the Stroop color word test (SCWT). Neurol. Sci. 37, 365–372. doi: 10.1007/s10072-015-2428-2
Caffarra, P., Vezzaini, G., Dieci, F., Zonato, F., and Venneri, A. (2002). Una versione abbreviata del test di Stroop: dati normativi nella popolazione italiana. Nuova Rivis. Neurol. 12, 111–115.
Campanholo, K. R., Romão, M. A., Machado, M. A. D. R., Serrao, V. T., Coutinho, D. G. C., Benute, G. R. G., et al. (2014). Performance of an adult Brazilian sample on the Trail Making Test and Stroop Test. Dement. Neuropsychol. 8, 26–31. doi: 10.1590/S1980-57642014DN81000005
Chafetz, M. D., and Matthews, L. H. (2004). A new interference score for the Stroop test. Arch. Clin. Neuropsychol. 19, 555–567. doi: 10.1016/j.acn.2003.08.004
Delis, D. C., Kaplan, E., and Kramer, J. H. (2001). Delis-Kaplan Executive Function System (D-KEFS) . San Antonio, TX: Psychological Corporation.
Djamshidian, A., O'Sullivan, S. S., Lees, A., and Averbeck, B. B. (2011). Stroop test performance in impulsive and non impulsive patients with Parkinson's disease. Parkinsonism Relat. Disord. 17, 212–214. doi: 10.1016/j.parkreldis.2010.12.014
Duncan, M. T. (2006). Assessment of normative data of Stroop test performance in a group of elementary school students Niterói. J. Bras. Psiquiatr. 55, 42–48. doi: 10.1590/S0047-20852006000100006
Fera, F., Nicoletti, G., Cerasa, A., Romeo, N., Gallo, O., Gioia, M. C., et al. (2007). Dopaminergic modulation of cognitive interference after pharmacological washout in Parkinson's disease. Brain Res. Bull. 74, 75–83. doi: 10.1016/j.brainresbull.2007.05.009
Gardner, R. W., Holzman, P. S., Klein, G. S., Linton, H. P., and Spence, D. P. (1959). Cognitive control: a study of individual consistencies in cognitive behaviour. Psychol. Issues 1, 1–186.
Golden, C. J. (1978). Stroop Color and Word Test: A Manual for Clinical and Experimental Uses . Chicago, IL: Stoelting Co.
Golden, C. J., and Freshwater, S. M. (2002). The Stroop Color and Word Test: A Manual for Clinical and Experimental Uses . Chicago, IL: Stoelting.
Hankee, L. D., Preis, S. R., Piers, R. J., Beiser, A. S., Devine, S. A., Liu, Y., et al. (2016). Population normative data for the CERAD word list and Victoria Stroop Test in younger-and middle-aged adults: cross-sectional analyses from the framingham heart study. Exp. Aging Res. 42, 315–328. doi: 10.1080/0361073X.2016.1191838
Hsieh, Y. H., Chen, K. J., Wang, C. C., and Lai, C. L. (2008). Cognitive and motor components of response speed in the Stroop test in Parkinson's disease patients. Kaohsiung J. Med. Sci. 24, 197–203. doi: 10.1016/S1607-551X(08)70117-7
Ingraham, L. J., Chard, F., Wood, M., and Mirsky, A. F. (1988). An Hebrew language version of the Stroop test. Percept. Mot. Skills 67, 187–192. doi: 10.2466/pms.1988.67.1.187
Ivnik, R. J., Malec, J. F., Smith, G. E., Tangalos, E. G., and Petersen, R. C. (1996). Neuropsychological tests' norms above age 55: COWAT, BNT, MAE token, WRAT-R reading, AMNART, STROOP, TMT, and JLO. Clin. Neuropsychol. 10, 262–278. doi: 10.1080/13854049608406689
Jankovic, J., McDermott, M., Carter, J., Gauthier, S., Goetz, C., Golbe, L., et al. (1990). Parkinson Study Group. Variable expression of Parkinson's disease: a base-line analysis of DATATOP cohort. Neurology 40, 1529–1534.
Jensen, A. R., and Rohwer, W. D. (1966). The Stroop Color-Word Test: a Review. Acta Psychol. 25, 36–93. doi: 10.1016/0001-6918(66)90004-7
PubMed Abstract | CrossRef Full Text
Kane, M. J., and Engle, R. W. (2003). Working-memory capacity and the control of attention: the contributions of goal neglect, response competition, and task set to Stroop interference. J. Exp. Psychol. Gen. 132, 47–70. doi: 10.1037/0096-3445.132.1.47
Kang, C., Lee, G. J., Yi, D., McPherson, S., Rogers, S., Tingus, K., et al. (2013). Normative data for healthy older adults and an abbreviated version of the Stroop test. Clin. Neuropsychol. 27, 276–289. doi: 10.1080/13854046.2012.742930
Lansbergen, M. M., Kenemans, J. L., and van Engeland, H. (2007). Stroop interference and attention-deficit/hyperactivity disorder: a review and meta-analysis. Neuropsychology 21:251. doi: 10.1037/0894-4105.21.2.251
Llinàs-Reglà, J., Vilalta-Franch, J., López-Pousa, S., Calvó-Perxas, L., and Garre-Olmo, J. (2013). Demographically adjusted norms for Catalan older adults on the Stroop Color and Word Test. Arch. Clin. Neuropsychol. 28, 282–296. doi: 10.1093/arclin/act003
Lopez, E., Salazar, X. F., Villasenor, T., Saucedo, C., and Pena, R. (2013). “Validez y datos normativos de las pruebas de nominación en personas con educación limitada,” in Poster Presented at The Congress of the “Sociedad Lationoamericana de Neuropsicologıa” (Montreal, QC).
Lubrini, G., Periañez, J. A., Rios-Lago, M., Viejo-Sobera, R., Ayesa-Arriola, R., Sanchez-Cubillo, I., et al. (2014). Clinical Spanish norms of the Stroop test for traumatic brain injury and schizophrenia. Span. J. Psychol. 17:E96. doi: 10.1017/sjp.2014.90
Lucas, J. A., Ivnik, R. J., Smith, G. E., Ferman, T. J., Willis, F. B., Petersen, R. C., et al. (2005). Mayo's older african americans normative studies: norms for boston naming test, controlled oral word association, category fluency, animal naming, token test, wrat-3 reading, trail making test, stroop test, and judgment of line orientation. Clin. Neuropsychol. 19, 243–269. doi: 10.1080/13854040590945337
MacLeod, C. M., and Dunbar, K. (1988). Training and Stroop-like interference: evidence for a continuum of automaticity. J. Exp. Psychol. Learn. Mem. Cogn. 14, 126–135. doi: 10.1037/0278-7393.14.1.126
McDowd, J. M., Oseas-Kreger, D. M., and Filion, D. L. (1995). “Inhibitory processes in cognition and aging,” in Interference and Inhibition in Cognition , eds F. N. Dempster and C. J. Brainerd (San Diego, CA: Academic Press), 363–400.
Google Scholar
Mitrushina, M., Boone, K. B., Razani, J., and D'Elia, L. F. (2005). Handbook of Normative Data for Neuropsychological Assessment . New York, NY: Oxford University Press.
Moering, R. G., Schinka, J. A., Mortimer, J. A., and Graves, A. B. (2004). Normative data for elderly African Americans for the Stroop color and word test. Arch. Clin. Neuropsychol. 19, 61–71. doi: 10.1093/arclin/19.1.61
Morrow, S. A. (2013). Normative data for the stroop color word test for a north american population. Can. J. Neurol. Sci. 40, 842–847. doi: 10.1017/S0317167100015997
Norman, M. A., Moore, D. J., Taylor, M., Franklin, D. Jr., Cysique, L., Ake, C., et al. (2011). Demographically corrected norms for African Americans and Caucasians on the hopkins verbal learning test–revised, brief visuospatial memory test–revised, stroop color and word test, and wisconsin card sorting test 64-card version. J. Clin. Exp. Neuropsychol. 33, 793–804. doi: 10.1080/13803395.2011.559157
Oliveira, R. M., Mograbi, D. C., Gabrig, I. A., and Charchat-Fichman, H. (2016). Normative data and evidence of validity for the Rey Auditory Verbal Learning Test, Verbal Fluency Test, and Stroop Test with Brazilian children. Psychol. Neurosci. 9, 54–67. doi: 10.1037/pne0000041
Peña-Casanova, J., Qui-ones-Ubeda, S., Gramunt-Fombuena, N., Quintana, M., Aguilar, M., Molinuevo, J. L., et al. (2009). Spanish multicenter normative studies (NEURONORMA Project): norms for the Stroop color-word interference test and the Tower of London-Drexel. Arch. Clin. Neuropsychol. 24, 413–429. doi: 10.1093/arclin/acp043
Rivera, D., Perrin, P. B., Stevens, L. F., Garza, M. T., Weil, C., Saracho, C. P., et al. (2015). Stroop color-word interference test: normative data for the Latin American Spanish speaking adult population. Neurorehabilitation 37, 591–624. doi: 10.3233/NRE-151281
Rognoni, T., Casals-Coll, M., Sánchez-Benavides, G., Quintana, M., Manero, R. M., Calvo, L., et al. (2013). Spanish normative studies in a young adult population (NEURONORMA young adults Project): norms for the Boston Naming Test and the Token Test. Neurología 28, 73–80. doi: 10.1016/j.nrl.2012.02.009
Rosselli, M., Ardila, A., Santisi, M. N., Arecco Mdel, R., Salvatierra, J., Conde, A., et al. (2002). Stroop effect in Spanish–English bilinguals. J. Int. Neuropsychol. Soc. 8, 819–827. doi: 10.1017/S1355617702860106
Seo, E. H., Lee, D. Y., Kim, S. G., Kim, K. W., Youn, J. C., Jhoo, J. H., et al. (2008). Normative study of the Stroop Color and Word Test in an educationally diverse elderly population. Int. J. Geriatr. Psychiatry 23, 1020–1027 doi: 10.1002/gps.2027
Shum, D. H. K., McFarland, K. A., and Brain, J. D. (1990). Construct validity of eight tests of attention: comparison of normal and closed head injured samples. Clin. Neuropsychol. 4, 151–162. doi: 10.1080/13854049008401508
Stacy, M., and Jankovic, J. (1992). Differential diagnosis of parkinson's disease and the parkinsonism plus syndrome. Neurol. Clin. 10, 341–359.
Steinberg, B. A., Bieliauskas, L. A., Smith, G. E., and Ivnik, R. J. (2005). Mayo's older Americans normative studies: age-and IQ-adjusted norms for the trail-making test, the stroop test, and MAE controlled oral word association test. Clin. Neuropsychol. 19, 329–377. doi: 10.1080/13854040590945210
Strauss, E., Sherman, E. M., and Spreen, O. (2006a). A Compendium of Neuropsychological Tests: Administration, Norms, and Commentary . Oxford: American Chemical Society.
Strauss, E., Sherman, E. M. S., and Spreen, O. (2006b). A Compendium of Neuropsychological Tests, 3rd Edn. New York, NY: Oxford University Press.
Strickland, T. L., D'Elia, L. F., James, R., and Stein, R. (1997). Stroop color-word performance of African Americans. Clin. Neuropsychol. 11, 87–90. doi: 10.1080/13854049708407034
Stroop, J. R. (1935). Studies of interference in serial verbal reactions. J. Exp. Psychol. 18, 643–662. doi: 10.1037/h0054651
Stuss, D. T., Floden, D., Alexander, M. P., Levine, B., and Katz, D. (2001). Stroop performance in focal lesion patients: dissociation of processes and frontal lobe lesion location. Neuropsychologia 39, 771–786. doi: 10.1016/S0028-3932(01)00013-6
Swick, D., and Jovanovic, J. (2002). Anterior cingulate cortex and the Stroop task: neuropsychological evidence for topographic specificity. Neuropsychologia 40, 1240–1253. doi: 10.1016/S0028-3932(01)00226-3
Tremblay, M. P., Potvin, O., Belleville, S., Bier, N., Gagnon, L., Blanchet, S., et al. (2016). The victoria stroop test: normative data in Quebec-French adults and elderly. Arch. Clin. Neuropsychol. 31, 926–933. doi: 10.1093/arclin/acw029
Trenerry, M. R., Crosson, B., DeBoe, J., and Leber, W. R. (1989). Stroop Neuropsychological Screening Test . Odessa, FL: Psychological Assessment Resources.
Troyer, A. K., Leach, L., and Strauss, E. (2006). Aging and response inhibition: normative data for the Victoria Stroop Test. Aging Neuropsychol. Cogn. 13, 20–35. doi: 10.1080/138255890968187
Valgimigli, S., Padovani, R., Budriesi, C., Leone, M. E., Lugli, D., and Nichelli, P. (2010). The Stroop test: a normative Italian study on a paper version for clinical use. G. Ital. Psicol. 37, 945–956. doi: 10.1421/33435
Van der Elst, W., Van Boxtel, M. P., Van Breukelen, G. J., and Jolles, J. (2006). The Stroop Color-Word Test influence of age, sex, and education; and normative data for a large sample across the adult age range. Assessment 13, 62–79. doi: 10.1177/1073191105283427
Vendrell, P., Junqué, C., Pujol, J., Jurado, M. A., Molet, J., and Grafman, J. (1995). The role of prefrontal regions in the Stroop task. Neuropsychologia 33, 341–352. doi: 10.1016/0028-3932(94)00116-7
Venneri, A., Molinari, M. A., Pentore, R., Cotticelli, B., Nichelli, P., and Caffarra, P. (1992). Shortened Stroop color-word test: its application in normal aging and Alzheimer's disease. Neurobiol. Aging 13, S3–S4. doi: 10.1016/0197-4580(92)90135-K
CrossRef Full Text
Waldrop-Valverde, D., Ownby, R. L., Jones, D. L., Sharma, S., Nehra, R., Kumar, A. M., et al. (2015). Neuropsychological test performance among healthy persons in northern India: development of normative data. J. Neurovirol. 21, 433–438. doi: 10.1007/s13365-015-0332-4
Zajano, M. J., and Gorman, A. (1986). Stroop interference as a function of percentage of congruent items. Percept. Mot. Skills 63, 1087–1096. doi: 10.2466/pms.1986.63.3.1087
Zalonis, I., Christidi, F., Bonakis, A., Kararizou, E., Triantafyllou, N. I., Paraskevas, G., et al. (2009). The stroop effect in Greek healthy population: normative data for the Stroop Neuropsychological Screening Test. Arch. Clin. Neuropsychol. 24, 81–88. doi: 10.1093/arclin/acp011
Zimmermann, N., Cardoso, C. D. O., Trentini, C. M., Grassi-Oliveira, R., and Fonseca, R. P. (2015). Brazilian preliminary norms and investigation of age and education effects on the Modified Wisconsin Card Sorting Test, Stroop Color and Word test and Digit Span test in adults. Dement. Neuropsychol. 9, 120–127. doi: 10.1590/1980-57642015DN92000006
Keywords: stroop color and word test, neuropsychological assessment, inhibition, executive functions, systematic review
Citation: Scarpina F and Tagini S (2017) The Stroop Color and Word Test Front. Psychol. 8:557. doi: 10.3389/fpsyg.2017.00557
Received: 10 November 2016; Accepted: 27 March 2017; Published: 12 April 2017.
Reviewed by:
Copyright © 2017 Scarpina and Tagini. This is an open-access article distributed under the terms of the Creative Commons Attribution License (CC BY) . The use, distribution or reproduction in other forums is permitted, provided the original author(s) or licensor are credited and that the original publication in this journal is cited, in accordance with accepted academic practice. No use, distribution or reproduction is permitted which does not comply with these terms.
*Correspondence: Federica Scarpina, [email protected]
Disclaimer: All claims expressed in this article are solely those of the authors and do not necessarily represent those of their affiliated organizations, or those of the publisher, the editors and the reviewers. Any product that may be evaluated in this article or claim that may be made by its manufacturer is not guaranteed or endorsed by the publisher.
Stroop Effect Experiment in Psychology
Charlotte Ruhl
Research Assistant & Psychology Graduate
BA (Hons) Psychology, Harvard University
Charlotte Ruhl, a psychology graduate from Harvard College, boasts over six years of research experience in clinical and social psychology. During her tenure at Harvard, she contributed to the Decision Science Lab, administering numerous studies in behavioral economics and social psychology.
Learn about our Editorial Process
Saul Mcleod, PhD
Editor-in-Chief for Simply Psychology
BSc (Hons) Psychology, MRes, PhD, University of Manchester
Saul Mcleod, PhD., is a qualified psychology teacher with over 18 years of experience in further and higher education. He has been published in peer-reviewed journals, including the Journal of Clinical Psychology.
On This Page:
The Stroop effect is a psychological phenomenon demonstrating interference in reaction time of a task. It occurs when the name of a color is printed in a color not denoted by the name, making it difficult for participants to identify the color of the word quickly and accurately.
Take-home Messages
- In psychology, the Stroop effect is the delay in reaction time between automatic and controlled processing of information, in which the names of words interfere with the ability to name the color of ink used to print the words.
- The Stroop test requires individuals to view a list of words printed in a different color than the word’s meaning. Participants are tasked with naming the color of the word, not the word itself, as fast as they can.
- For example, when presented with the word “green” written in red ink, it is much easier to name the word that is spelled instead of the color ink in which the word is written.
- The interference, or the delay in response time, is measured by comparing results from the conflict condition (word and color mismatch) to a neutral condition (e.g., a block of color or a color word with matching ink). Subtracting the results from these two conditions helps to eliminate the influence of general motor responses.
- Reading, a more powerful automatic process, takes some precedence over color naming, which requires higher cognitive demands.
- Since psychologist John Ridley Stroop first developed this paradigm back in 1935, the Stroop task has since been modified to help understand additional brain mechanisms and expanded to aid in brain damage and psychopathology research.
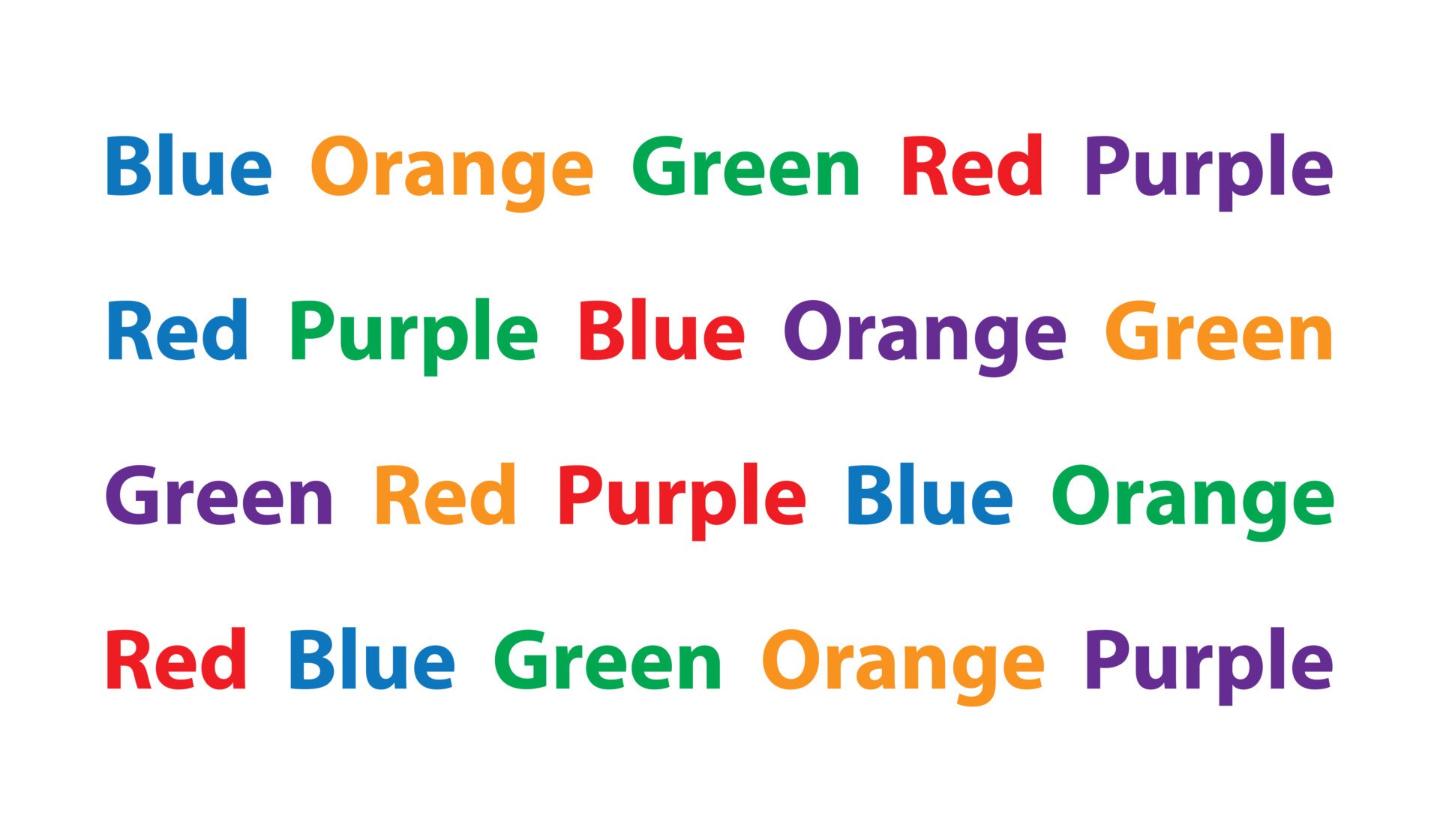
What Is The Stroop Effect?
The Stroop effect refers to a delay in reaction times between congruent and incongruent stimuli (MacLeod, 1991).
Congruency, or agreement, occurs when a word’s meaning and font color are the same. For example, if the word “green” is printed in green.
Incongruent stimuli are just the opposite. That is the word’s meaning and the color in which it is written do not align. For example, the word “green” might be printed in red ink.
The Stroop task asks individuals to name the color of the word instead of reading the word itself.
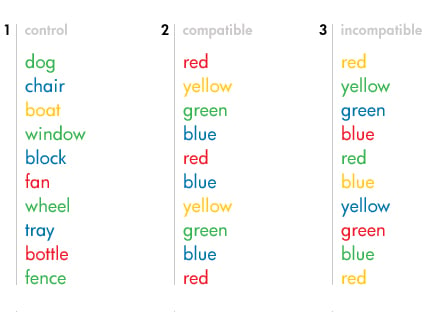
The delay in reaction time reveals that it is much harder to name the color of a word when the word itself spells another color (the incongruent stimuli) than it is to name the color of the word when the word itself spells that same color (the congruent stimuli).
The First Stroop Experiment
The Stroop effect was first published in 1935 by American psychologist John Ridley Stroop, although discoveries of this phenomenon date back to the nineteenth century (Stroop, 1935).
Building off previous research, Stroop had two main aims in his groundbreaking paper:
- To examine how incongruency between the color of the word and the word’s content will impair the ability to name the color.
- To measure what effect practicing reacting to color stimuli in the presence of conflicting word stimuli would have upon the reaction times.
To empirically study these two major aims, Stroop ran three different experiments:
1) Experiment 1 :
Participants (70 college undergraduates) were tasked with reading the word aloud, irrespective of its color. In other words, participants must read aloud the word “green” even if written in a different color.
2) Experiment 2 :
The second experiment was the opposite of the first. Participants (100 college students) were first asked to name the color of individual squares (instead of the color of words) as a training mechanism for the subsequent task. Afterward, participants had to say the color of the word, regardless of its meaning – the opposite of the experiment 1 procedure.
3) Experiment 3 :
The third and final experiment integrated all of the previously mentioned tests with an undergraduate population of 32 participants.
The independent variable (IV) was the congruency of the font name and color.
- Congruent (word name and font color are the same)
- Incongruent (word name and font color are different)
The dependent variable (DV) was reaction time (ms) in reporting the letter color.
After running the three experiments, Stroop drew two main conclusions:
- The interference of conflicting word stimuli upon the time for naming colors caused an increase of 47.0 seconds or 74.3 percent of the normal time for naming colors printed in just squares.
- The interference of conflicting color stimuli upon the time for reading words caused an increase of only 2.3 seconds or 5.6 percent over the normal time for reading the same words printed in black.
These tests demonstrate a disparity in the speed of naming colors and reading the names of colors, which may be explained by a difference in training in the two activities.
The word stimulus has been associated with the specific response “to read,” while the color stimulus has been associated with various responses: “to admire,” “to name,” etc.
The observed results might reflect the fact that people have more experience consciously reading words than consciously labeling colors, illustrating a difference in the mechanisms that control these two processes.
How the Stroop Effect Works
Why does the Stroop effect occur? We can tell our brain to do lots of things – store memories, sleep, think, etc. – so why can’t we tell it to do something as easy as naming a color? Isn’t that something we learn to do at a very young age?
Researchers have analyzed this question and come up with multiple different theories that seek to explain the occurrence of the Stroop effect (Sahinoglu & Dogan, 2016).
Speed of processing theory:
The processing speed theory claims that people can read words much faster than they can name colors (i.e., word processing is much faster than color processing).
When we look at the incongruent stimuli (the word “green” printed in red, for example), our brain first reads the word, making it much more difficult to then have to name the color.
As a result, a delay occurs when trying to name the color because doing so is not our brain’s first instinct (McMahon, 2013).
Selective attention theory:
The theory of selective attention holds that recognizing colors, compared to reading words, requires more attention.
Because of this, the brain needs to use more attention when attempting to name a color, making this process take slightly longer (McMahon, 2013).
Automaticity:
A prevalent explanation for the Stroop effect is the automatic nature of reading. When we see a word, its meaning is almost instantly recognized. Thus, when presented with a conflicting color, there’s interference between the automatic reading process and the task of naming the ink color.
This theory argues that recognizing colors is not an automatic process , and thus there is a slight hesitancy when carrying out this action.
Automatic processing is processed in the mind that is relatively fast and requires few cognitive resources.
This type of information processing generally occurs outside of conscious awareness and is common when undertaking familiar and highly practiced tasks.
However, the brain is able to automatically understand the meaning of a word as a result of habitual reading (think back to Stroop’s initial study in 1935 – this theory explains why he wanted to test the effects of practice on the ability to name colors).
Word reading, being more automatic and faster than color naming, results in involuntary intrusions during the color-naming task. Conversely, reading isn’t affected by the conflicting print color.
Researchers in support of this theory posit that automatic reading does not need controlled attention but still uses enough of the brain’s attentional resources to reduce the amount left for color processing (Monahan, 2001).
In a way, this parallels the brain’s dueling modes of thinking – that of “System 1” and “System 2.” Whereas the former is more automatic and instinctive, the latter is slower and more controlled (Kahneman, 2011).
This is similar to the Stroop effect, in which we see a more automatic process trying to dominate over a more deliberative one. The interference occurs when we try to use System 2 to override System 1, thus producing that delay in reaction time.
Parallel distributed processing:
The fourth and final theory proposes that unique pathways are developed when the brain completes different tasks. Some of these pathways, such as reading words, are stronger than others, such as naming colors (Cohen et al., 1990).
Thus, interference is not an issue of processing speed, attention, or automaticity but rather a battle between the stronger and weaker neural pathways.
Additional Research
John Ridley Stroop helped lay the groundwork for future research in this field.
Numerous studies have tried to identify the specific brain regions responsible for this phenomenon, identifying two key regions: the anterior cingulate cortex (ACC) and dorsolateral prefrontal cortex (DLFPC).
Both MRI and fMRI scans show activity in the ACC and DLPFC while completing the Stroop test or related tasks (Milham et al., 2003).
The DLPFC assists with memory and executive functioning, and its role during the task are to activate color perception and inhibit word encoding. The ACC is responsible for selecting the appropriate response and properly allocating attentional resources (Banich et al., 2000).
Countless studies that repeatedly test the Stroop effect reveal a few key recurring findings (van Maanen et al., 2009):
- Semantic interference : Naming the ink color of neutral stimuli (where the color is only shown in blocks, not as a written word) is faster than incongruent stimuli (where the word differs from its printed color).
- Semantic facilitation : Naming the ink of congruent stimuli (where the word and its printed color are in agreement) is faster than for neutral stimuli.
- Stroop asynchrony : The previous two findings disappear when reading the word, not naming the color, is the task at hand – supporting the claim that it is much more automatic to read words than to name colors.
Other experiments have slightly modified the original Stroop test paradigm to provide additional findings.
One study found that participants were slower to name the color of emotion words as opposed to neutral words (Larsen et al., 2006).
Another experiment examined the differences between participants with panic disorder and OCD. Even with using threat words as stimuli, they found that there was no difference among panic disorder, OCD, and neutral participants’ ability to process colors (Kampman et al., 2002).
A third experiment investigated the relationship between duration and numerosity processing instead of word and color processing.
Participants were shown two series of dots in succession and asked either (1) which series contained more dots or (2) which series lasted longer from the appearance of the first to the last dots of the series.
The incongruency occurred when fewer dots were shown on the screen for longer, and a congruent series was marked by a series with more dots that lasted longer.
The researchers found that numerical cues interfered with duration processing. That is, when fewer dots were shown for longer, it was harder for participants to figure out which set of dots appeared on the screen for longer (Dormal et al., 2006).
Thus, there is a difference between the processing of numerosity and duration. Together, these experiments illustrate not only all of the doors of research that Stroop’s initial work opened but also shed light on all of the intricate processing associations that occur in our brains.
Other Uses and Versions
The purpose of the Stroop task is to measure interference that occurs in the brain. The initial paradigm has since been adopted in several different ways to measure other forms of interference (such as duration and numerosity, as mentioned earlier).
Additional variations measure interference between picture and word processing, direction and word processing, digit and numerosity processing, and central vs. peripheral letter identification (MacLeod, 2015).
The below figure provides illustrations for these four variations:
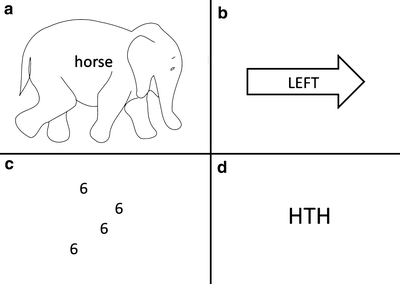
The Stroop task is also used as a mechanism for measuring selective attention, processing speed, and cognitive flexibility (Howieson et al., 2004).
The Stroop task has also been utilized to study populations with brain damage or mental disorders, such as dementia, depression, or ADHD (Lansbergen et al., 2007; Spreen & Strauss, 1998).
For individuals with depression, an emotional Stroop task (where negative words, such as “grief,” “violence,” and “pain,” are used in conjunction with more neutral words, such as “clock,” “door,” and “shoe”) has been developed.
Research reveals that individuals who struggle with depression are more likely to say the color of a negative word slower than that of a neutral word (Frings et al., 2010).
The versatility of the Stroop task paradigm lends itself to be useful in a wide variety of fields within psychology. What was once a test that only examined the relationship between word and color processing has since been expanded to investigate additional processing interferences and to contribute to the fields of psychopathology and brain damage.
The development of the Stroop task not only provides novel insights into the ways in which our brain mechanisms operate but also sheds light on the power of psychology to expand and build on past research methods as we continue to uncover more and more about ourselves.
Critical Evaluation
Dishon-Berkovits and Algom (2000) argue that the Stroop effect is not a result of automatic processes but is due to incidental correlations between the word and its color across stimuli.
They suggest that participants unconsciously recognize these correlations, using word cues to anticipate the correct color hue they should name.
When testing with word-word stimuli, Dishon-Berkovits and Algom created positive, negative, and zero correlations.
They observed that zero correlations nearly eliminated Stroop effects, implying that the effects might be more about the way stimuli are presented rather than true indicators of automaticity or attention.
However, their methodology raised concerns:
- They had difficulty creating zero correlations with color-hue situations.
- Their study didn’t include a neutral condition, which means interference and facilitation were not examined.
- There’s a general finding that facilitation effects are smaller than interference effects, which their findings don’t necessarily support
Despite these considerations, the correlational approach does not invalidate Stroop’s original paradigm or the many studies based on it.
Stroop-based findings have been instrumental in understanding various clinical conditions like anxiety, schizophrenia, ADHD, dyslexia, PTSD, racial attributions, and others.
The takeaway is that while the theory proposed by Dishon-Berkovits and Algom introduces a fresh perspective, it does not negate the established findings and implications of the Stroop effect.
Instead, it encourages a deeper examination of how automaticity and attention might be influenced by certain environmental factors and correlations.
Describe why the Stroop test is challenging for us.
The Stroop test is challenging due to the cognitive conflict it creates between two mental processes: reading and color recognition. Reading is a well-learned, automatic process, whereas color recognition requires more cognitive effort.
When the word’s color and its semantic meaning don’t match, our brain’s automatic response to reading the word interferes with naming the color, causing a delay in response time and an increase in mistakes. This is known as the Stroop effect.
Banich, M. T., Milham, M. P., Atchley, R., Cohen, N. J., Webb, A., Wszalek, T., … & Magin, R. (2000). fMRI studies of Stroop tasks reveal unique roles of anterior and posterior brain systems in attentional selection . Journal of cognitive neuroscience, 12 (6), 988-1000.
Cohen, J. D., Dunbar, K., & McClelland, J. L. (1990). On the control of automatic processes: a parallel distributed processing account of the Stroop effect . Psychological Review, 97 (3), 332.
Dishon-Berkovits, M., & Algom, D. (2000). The Stroop effect: It is not the robust phenomenon that you have thought it to be . Memory & Cognition , 28 , 1437-1449.
Dormal, V., Seron, X., & Pesenti, M. (2006). Numerosity-duration interference: A Stroop experiment . Acta psychologica, 121 (2), 109-124.
Frings, C., Englert, J., Wentura, D., & Bermeitinger, C. (2010). Decomposing the emotional Stroop effect . Quarterly journal of experimental psychology, 63 (1), 42-49.
Howieson, D. B., Lezak, M. D., & Loring, D. W. (2004). Orientation and attention. Neuropsychological assessment , 365-367.
Kahneman, D. (2011). Thinking, fast and slow . Macmillan.
Kampman, M., Keijsers, G. P., Verbraak, M. J., Näring, G., & Hoogduin, C. A. (2002). The emotional Stroop: a comparison of panic disorder patients, obsessive–compulsive patients, and normal controls, in two experiments. Journal of anxiety disorders, 16 (4), 425-441.
Lansbergen, M. M., Kenemans, J. L., & Van Engeland, H. (2007). Stroop interference and attention-deficit/hyperactivity disorder: a review and meta-analysis . Neuropsychology, 21 (2), 251.
Larsen, R. J., Mercer, K. A., & Balota, D. A. (2006). Lexical characteristics of words used in emotional Stroop experiments . Emotion, 6 (1), 62.
MacLeod, C. M. (1991). Half a century of research on the Stroop effect: an integrative review . Psychological bulletin, 109 (2), 163.
MacLeod, C. M. (2015). The stroop effect. Encyclopedia of Color Science and Technology.
McMahon, M. (2013). What Is the Stroop Effect. Retrieved November, 11 .
Milham, M. P., Banich, M. T., Claus, E. D., & Cohen, N. J. (2003). Practice-related effects demonstrate complementary roles of anterior cingulate and prefrontal cortices in attentional control . Neuroimage, 18 (2), 483-493.
Monahan, J. S. (2001). Coloring single Stroop elements: Reducing automaticity or slowing color processing? . The Journal of general psychology, 128 (1), 98-112.
Sahinoglu B, Dogan G. (2016). Event-Related Potentials and the Stroop Effect. Eurasian J Med , 48(1), 53‐57.
Spreen, O., & Strauss, E. (1998). A compendium of neuropsychological tests: Administration, norms, and commentary . Oxford University Press.
Stroop, J. R. (1935). Studies of interference in serial verbal reactions . Journal of experimental psychology, 18 (6), 643.
van Maanen, L., van Rijn, H., & Borst, J. P. (2009). Stroop and picture—word interference are two sides of the same coin . Psychonomic bulletin & review, 16 (6), 987-999.
Further information
- Exampe of a stroop effect lab report
- Picture-word interference is a Stroop effect: A theoretical analysis and new empirical findings

Related Articles
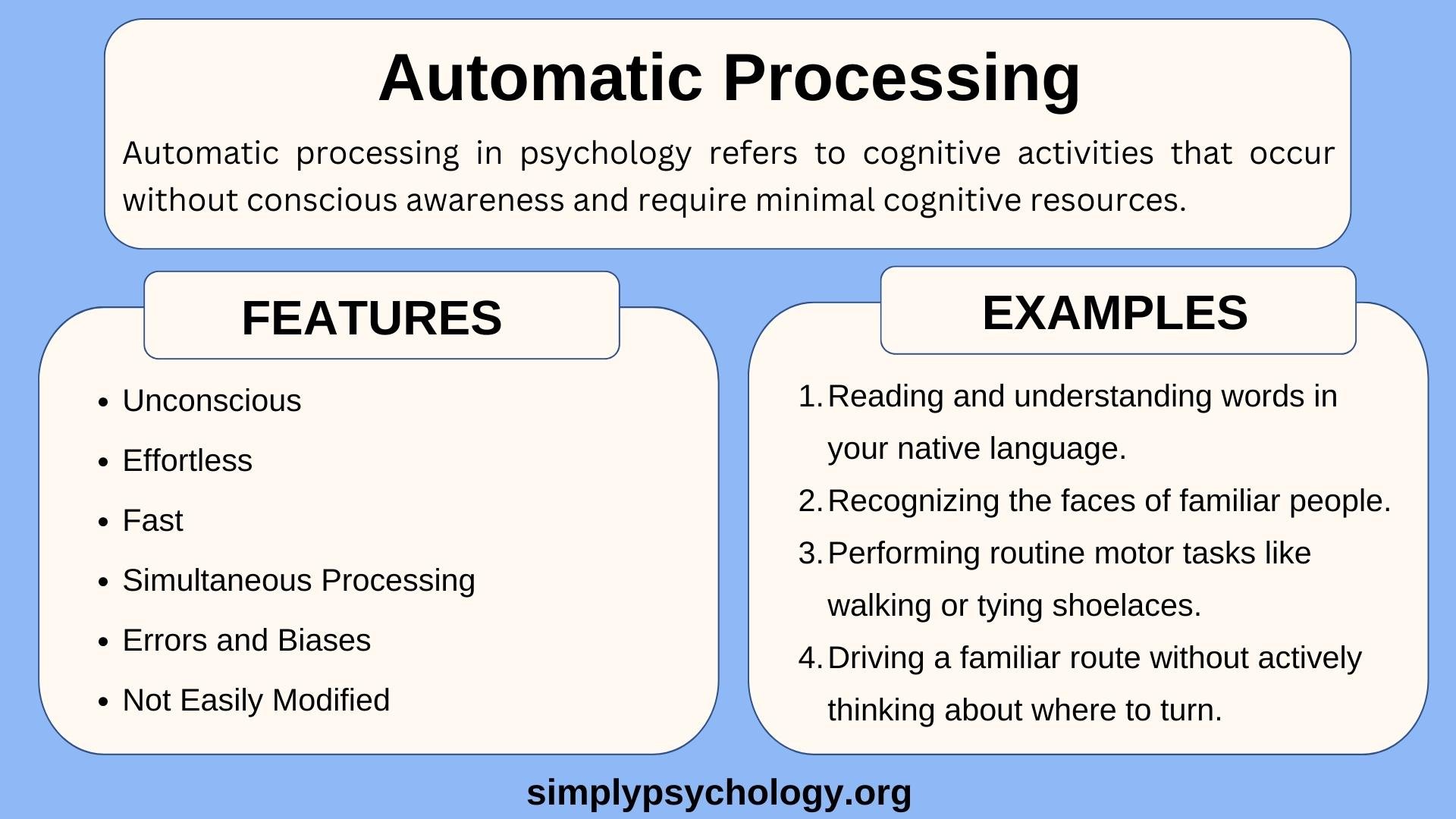
Cognitive Psychology
Automatic Processing in Psychology: Definition & Examples
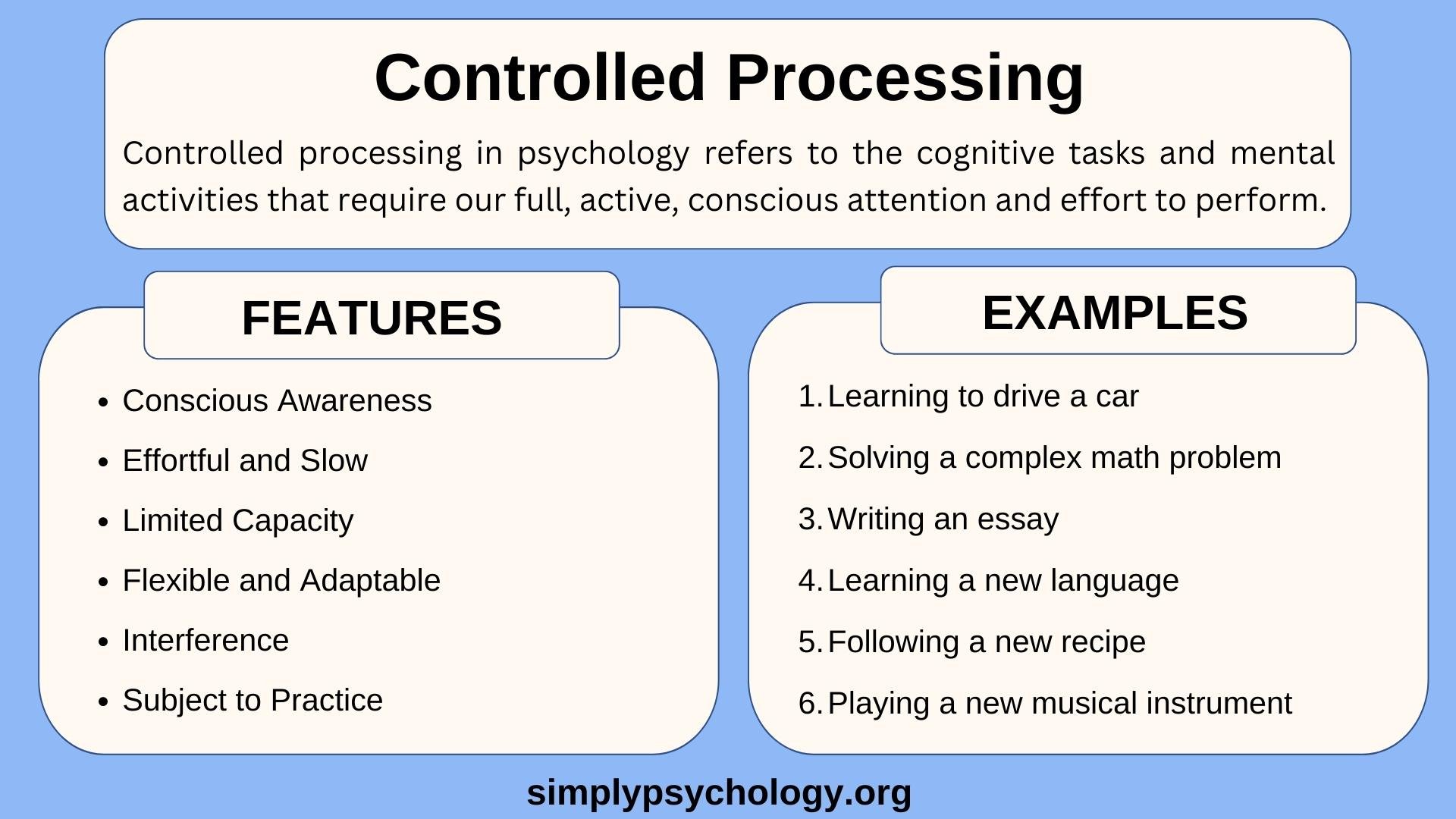
Controlled Processing in Psychology: Definition & Examples
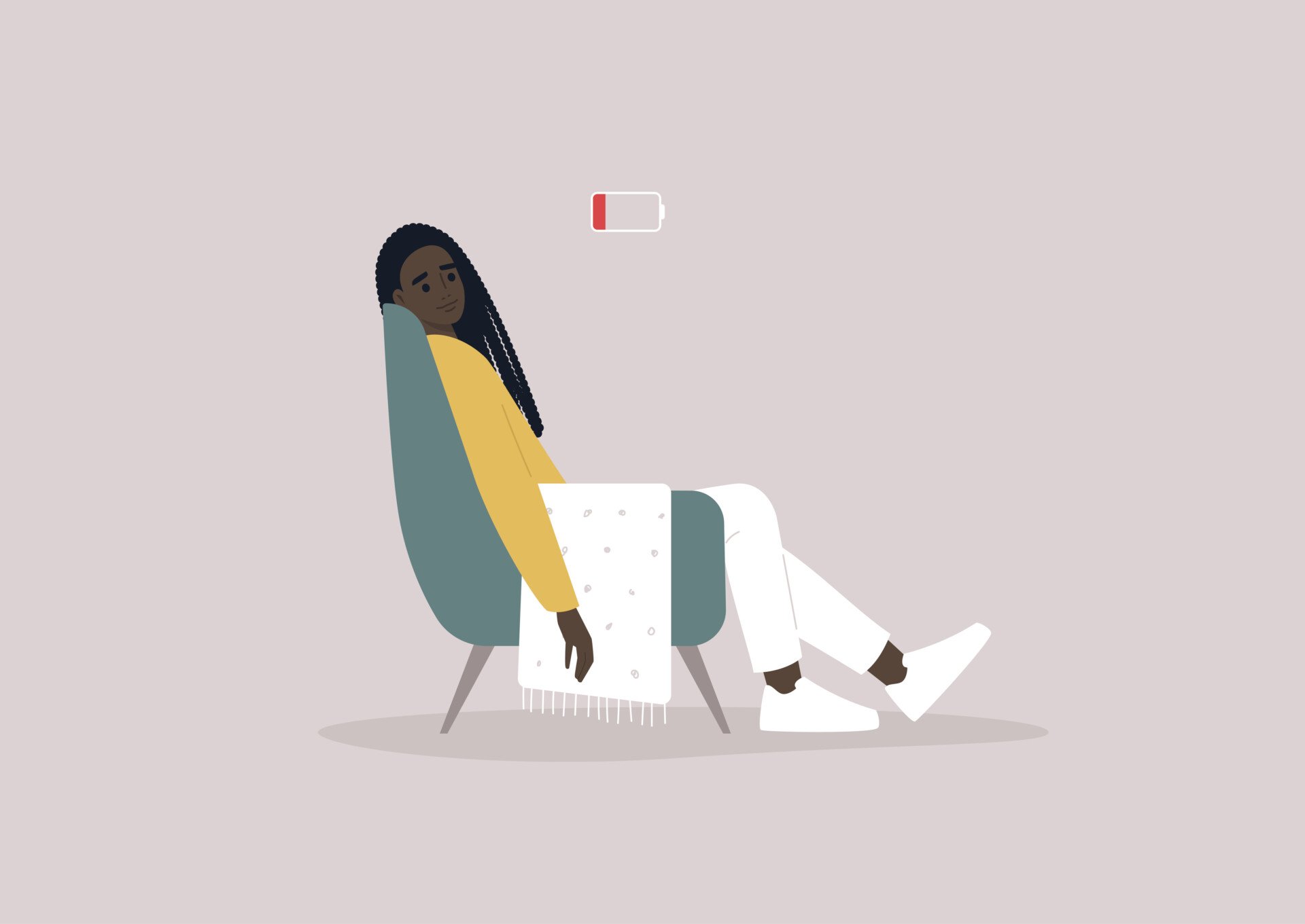
How Ego Depletion Can Drain Your Willpower
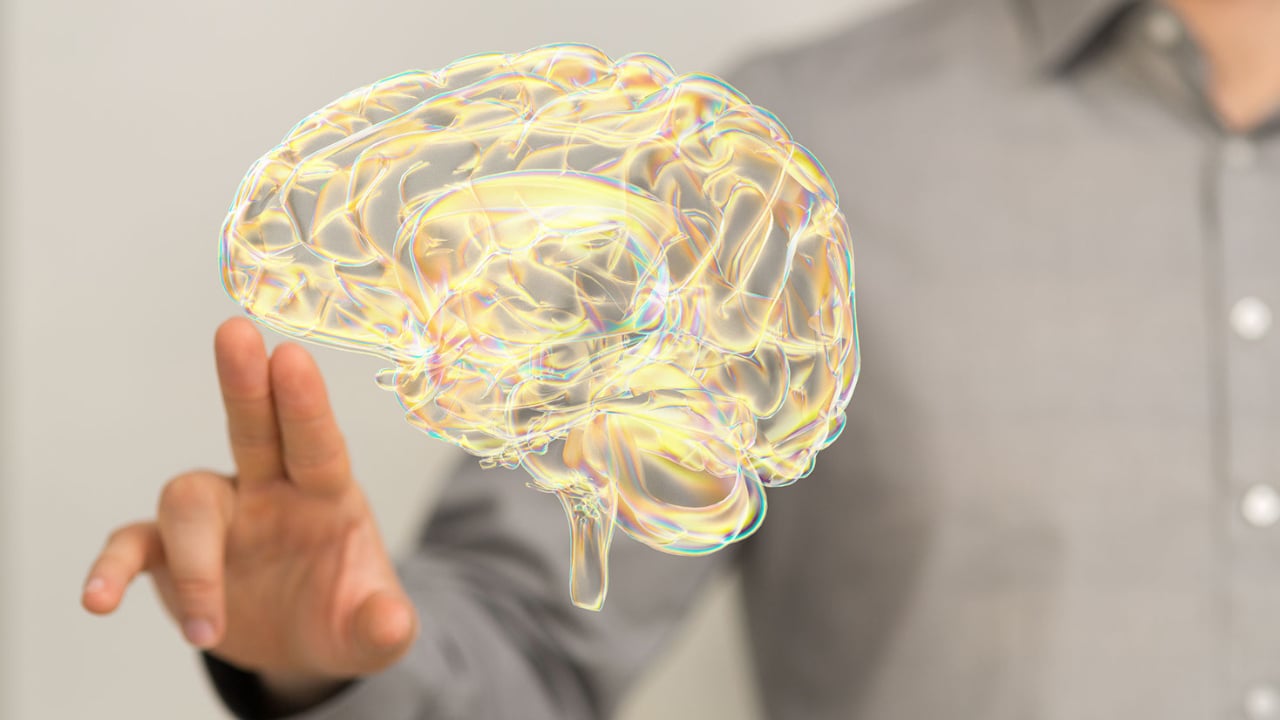
What is the Default Mode Network?
Theories of Selective Attention in Psychology
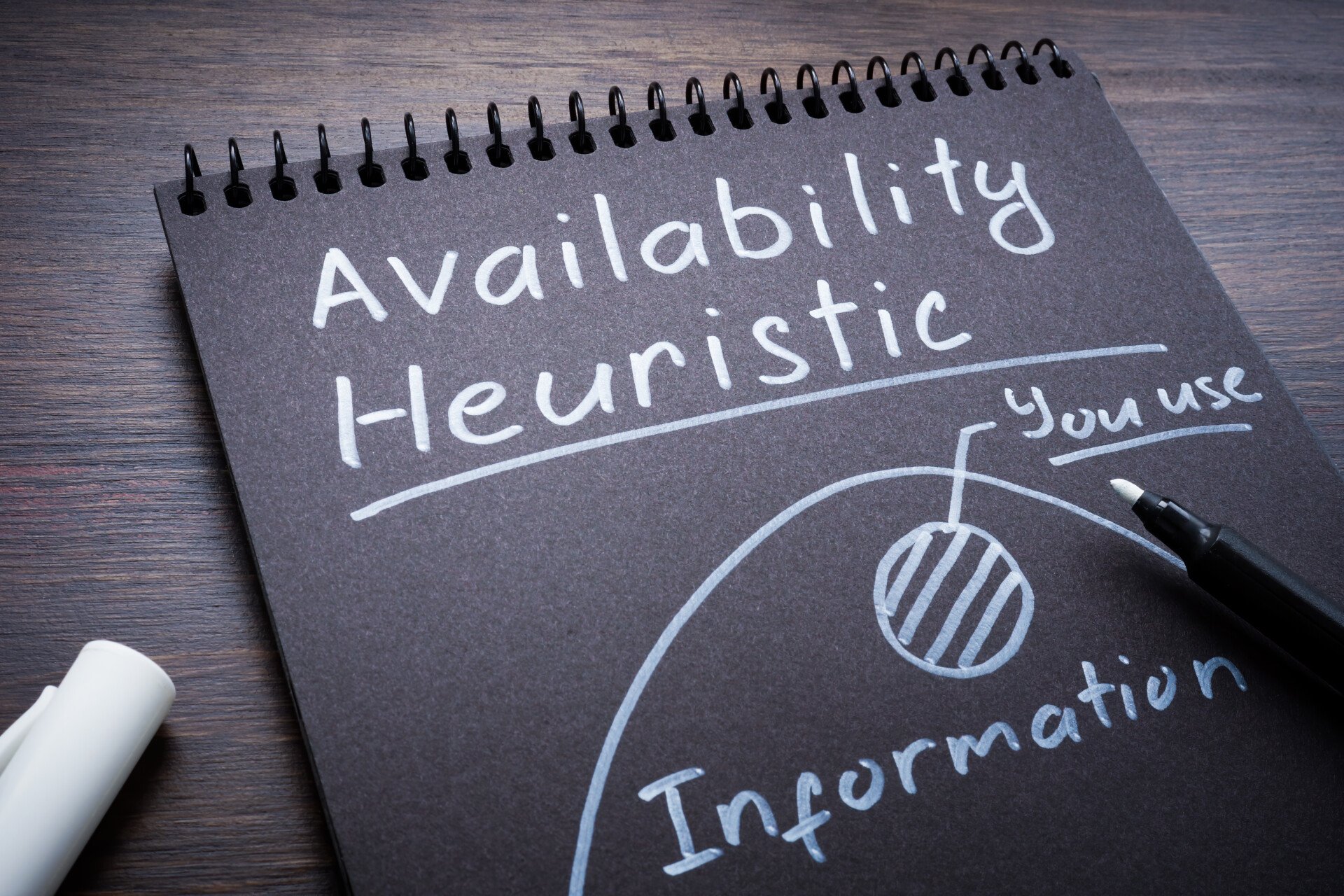
Availability Heuristic and Decision Making
Experiment in Cognition: Stroop Effect Research Paper
Introduction.
The current research paper is aimed at investigating the effect of interference in cognitive processes, which is known under the title of the Stroop effect. The study introduces the overview of the research conducted on the topic within a century of scientific work in the field of psychology. A variety of modifications of the experiment are discussed, and the theoretical explanations behind the investigated cognitive effect are explicitly articulated. The method section depicts the specifics of the experimental design, including the characteristics of the participants, the materials used, and the procedure. The results are demonstrated in the form of a table that demonstrates the descriptive statistics of the experiment. Finally, the findings are discussed to validate the hypothesis and explain the theory behind the identified cognitive effect.
The human cognitive processes have long been the focus of psychological research. The way the brain processes different kinds of information affects the psychological and emotional spheres of individuals’ lives and constitutes a relevant research topic. The issues of people’s abilities to multitask and manage some physical or cognitive processes automatically present a number of research cases for psychologists. The patterns and specific features of brain work in general, and the functions of attention in particular, are at the center of scientists’ attention. One such pattern is the Stroop effect named after an American psychologist of the first half of the twentieth century who introduced the phenomenon of cognitive interference to the field of neuropsychology. In this research paper, the fundamentals of the Stroop effect, as they are presented by Stroop (1935), will be used as a basis for an experiment investigating the impact of cognitive interference on the time of response to stimuli.
The experiment conducted within the realm of this research study is a modification of the original experiment presented by Stroop (1935) in his article “Studies of interference in serial verbal reactions.” The work by Stroop (1935) was comprised of three similar experiments. The first one required reading the names of the colors when the ink color was different from the one named in the card. The second one asked the participants to name the color of the ink in which the words were typed under the circumstances where the colors named and the inked used for typing the same card were different. The third experiment investigated how practice influenced the time of response to interfering stimuli. Consequently, the results showed that the time spent on naming colors of the ink was 74 percent longer than that spent on reading the names (Stroop, 1935, p. 651). However, practice showed to have a positive effect on decreasing the time of reaction, implying the training implications of the cognitive processes.
The experiment discussed above became a significant benchmark in cognitive psychology that brought light onto the very important issues of cognition particularities. It incepted the phenomenon called the Stroop effect, meaning the delay in response to incongruent stimuli that explains the basic principles of how the human brain disseminates attention and processes information. Many researchers in the fields of neuropsychology, emotional psychology, and other branches of science continue to refer to the Stroop effect. Several variations have entered the scholarly circles since the first publication of Stroop’s (1935) article. Much investigation has been made to expand the clinical, theoretical, and scientific implications of the issue. Indeed, according to MacLeod (1991), such psychologists as McCown and Arnoult, Regan, Kipnis and Glickman, Golden, and others have significantly modified the original experiment by changing the conditions of the process, the stimuli, or the presentation of the word. In such a manner, they attempted to measure more complex issues related to interference. The Stroop effect contributes to the studying of individual differences in cognition, mental conditions, the flexibility of attention, and other psychological particularities.
Today, the Stroop task is very well-known and is applied in both clinical settings and for educational or training purposes. Mainly it is used to test “the ability to inhibit cognitive interference, which occurs when the processing of a stimulus feature affects the simultaneous processing of another attribute of the same stimulus” (Scarpina & Tagini, 2017, p. 1). More variations continue to appear, where the semantics of the stimuli is altered to test emotional spheres, or the words are substituted with pictures, which allows obtaining more substantial results (MacLeod, 1991). However, the original test, which is the serial color-word test, remains highly relevant for testing cognitive abilities and the particularities of attention in individuals. Therefore, the standard version of the experiment is going to be carried out within this research study.
The experimental procedure allows for retrieving the exact information about the time spent by a participant in responding to the colors of the letter strings under different conditions. The difference in conditions is defined by either the absence or presence of interfering stimuli. As the academic literature on the issues suggests, the reason why participants spend more time naming the colors of letter strings under incongruent conditions is due to the automaticity of the reading process. Indeed, Stroop (1935), in his third experiment, came to the conclusion that practice influences performance in a positive way. The researcher stated that the differences in response delay when the subjects’ “habitual reaction pattern is interfering with reactions to a stimulus for which the subjects do not have a habitual reaction pattern” (Stroop, 1935, p. 658). In other words, people have an automatic habit of reading and understanding the meaning of the words but have to make an effort to name the colors since this process is not automatic.
This explanation refers to the reading habits and might be presented in a modified form. Indeed, the issues of the reading process automaticity are claimed by Megherbi, Elbro, Oakhill, Segui, and New (2017). The scholars investigated the Stroop effect in children whose reading skills are very low. The absence of the effect in such conditions proves the complexity of the cognitive processes that deal with decoding interfering stimuli when participating in the experiment. The researchers argue that this process contains two effects, including “the obligatory decoding of the distracting words” and the ability to “block out, to suppress, or inhibit the potential distraction” (Megherbi et al., 2017, p.657). Therefore, the delay in response to incongruent cards represents the multi-staged cognitive processes.
However, the neuropsychological perspective on the nature of the Stroop effect provides a more detailed explanation of the processes taking place in the human brain when completing the Stroop task. According to Banich (2019), there is a cascade-of-control model that includes four processes behind the Stroop effect. Each of them is processed in separate brain regions and deals with different goals necessary for task completion. Firstly, prefrontal and posterior brain regions come in conflict when processing the information that is either relevant or irrelevant for the task. Secondly, the mid regions of the brain process the working memory to include the information necessary for the task. Thirdly, separate areas of the brain are involved in giving responses in the later stages. Fourthly, “rostral dorsal regions of the anterior cingulate cortex … evaluate the appropriateness of the response selected and send feedback to lateral prefrontal regions to make adjustments in control as needed” (Banich, 2019, p. 2). Thus the inclusion of various loci of the brain to respond to inconsistent stimuli explains the existence of the Stroop effect.
All the above-mentioned theoretical explanations of the Stroop task implications allow for anticipating the results of the current research. Since the experiment will involve three different conditions, including neutral, consistent, and inconsistent, the response time will be different for each of them due to the level of interference between stimuli. The hypothesis for the current research is based on the literature review and helps to assume that the time spent on responding to inconsistent stimuli would be longer than the time spent on responding to consistent stimuli or neutral stimuli. Also, the response time under consistent conditions will be shorter than under neutral one due to the facilitating effect of color-word congruence. It is anticipated that the results of the study will be compatible with those obtained in the classical serial color-word experiment.
Participant
For the experiment held in a digital setting, it is sufficient to engage one participant to investigate the particularities of his or her cognition and attention. The participant in the current study is a healthy 24-year-old student at Athabasca University. The participant is a healthy individual without any physical or mental issues that might affect the results of the procedure. Before the beginning of the task, the student was instructed as per the conditions and rules relevant to the successful completion of the experiment. Also, the individual was informed that no personal information would be disclosed upon the procedure; only the results in the form of response time and errors will be collected. The participant has signed the informed consent form, thus agreeing to cooperate.
The experiment is conducted digitally with the utilization of electronic cards. Therefore, the materials used for the procedure include a computer and software that presents the experiment. The program contains three types of cards representing each of the three task conditions. The four colors used either as the ink color or as the words naming the colors are red, green, blue, and yellow. The first set of cards is aimed at testing responses in a neutral condition and contains colored letter strings in the form of Xs. The second type of cards includes those measuring interference and consists of the words the meanings of which do not match the ink color in which they are typed. The third set of cards is used to test a facilitating condition and presents the cards with words, meanings, and the ink color of which are consistent. Overall, there are 36 cards of each type, which are displayed twice; ultimately, a participant is asked to respond to 216 cards.
The procedure of the experiment is estimated to last for a maximum of thirty minutes. In the beginning, the participant is instructed according to the specifications of the experiment and its features. The informed consent is signed to retrieve the agreement for processing the information collected during the experiment. The task that is necessary to complete during the procedure is to name the color of the letter strings on the screen regardless of the meaning of the words typed in those colors. The colors used for the experiments are limited to four (red, green, blue, and yellow), each attributed to a particular button on the computer keyboard. More specifically, the red color is represented by ‘z’s, the green color is represented by ‘x’s, the blue color is represented by ‘.’, and the yellow color is represented by ‘/’. The symbols synchronized with the colors are displayed at the top of the screen throughout the whole experiment; however, it is advised to mark the respective buttons with colored patches for better recognition.
The participant is explained that it is required to respond to the color of the letters displayed on the screen by pressing a respective button as quickly as possible. Prior to the beginning of the test, a training session is run. Each card is shown on the screen for 1500 milliseconds, the pause between consecutive cards is represented by a white ‘x’s on the black screen and lasts for 500 milliseconds, after which the next card is shown. If the participant does not respond within the designated time or makes a mistake, a short sound is played, and the next card appears on the screen. Each set of cards (two sets containing 36 cards per one of the three conditions) is displayed randomly with pauses between them. The participant is obliged to press any button to start a new set of cards.
Upon the completion of the experiment, the results table is displayed that demonstrates the data retrieved during the procedure. Descriptive data portraying the time spent on each response, the code of the color, the text of a stimulus, and the type of conditions are available to review. Also, the summarized data showing the meantime of delay in response, standards deviation, and the number of trials (indicating errors) is presented. Finally, the group results collected from all participants taking part in the experiment and saved on a server are available for a researcher to compare the results of the participant with the average.
The participant has followed the instructions thoroughly and responded to each card attentively. The color patches were attached to the buttons of the keyboard for convenience. The pauses between different sets of cards were minimal. The summarized data is demonstrated in the form of descriptive statistics in Table 1 and shows the exact results of the task completion under three different conditions. The mean time of response is indicated in milliseconds spent per card. The standard deviation depicts the difference between the longest and the shortest reaction within the specific condition. The number of trials shows if there were errors made during the procedure.
As it is seen from the table, the neutral condition required more time to respond than the facilitation condition and let time than the interference condition. The interference condition or the one with the inconsistent representation of words and colors took the longest time of reaction. Finally, the facilitation condition or the one with the consistent representation of colors and words was the easiest task and took the least time to react. The number of errors (two) is insignificant and does not affect the results of the study. Overall, the results retrieved during the experiment on the Stroop effect are consistent with those demonstrating the average summarized data collected from 1476 participants. The group means time in condition 1 is 744.23, 809.65 in condition two, and 679.88 in condition three.
The current research hypothesized that the time spent on the responses under an inconsistent condition would be longer than that for responding under neutral or consistent conditions. The hypothesis was proved and showed that the dependent variable (the time of reaction) changed depending on the change in independent variables (colors of the words). The mean time for reacting to the stimuli where words and colors are different is 986.63. The time of reaction on the stimuli where the word meanings and the ink color match is 675.86. Therefore, the reaction to incongruent stimuli is delayed by 68.5 percent in comparison to the reaction to congruent word-color representation. These results match the initial findings made by Stroop (1935). As for the neutral condition where the participant had to name the color of letters X strings, the delay in the reaction was longer than in a facilitating condition.
To explain the Stroop effect identified within the conducted experiment, it is relevant to refer to the theory of automatic reading reaction and the complexity of the brain processes related to selective attention. In accordance with the claims made by Megherbi et al. (2017), reading is an automatic and uncontrolled reaction to words that occurs effortlessly and provokes an immediate reaction. However, the naming of the colors is not an automatic process; it requires a more complex analysis of information than involves blocking the data retrieved upon reading to respond according to the task requirements. Moreover, the fact that the reaction needs to be demonstrated not verbally, but by pressing a respective button, the brain takes time to validate the choice of the button. Therefore, the delay in the reaction under the inconsistent condition represents the Stroop effect. On the contrary, when the word is written in the color it names (for example, when the word ‘red’ is typed in red ink), which complies with the facilitating condition, the reaction is significantly faster since it is based solely on automatic reading.
The results of the experiment are valid and consistent with those obtained within the original study conducted by Stroop (1935). The indicators of reaction time might be used to interpret the individual cognitive characteristics of the participant. Nonetheless, the limitations of the research might include the engagement of only one participant, whose results were compared to those of a group. Also, the possible confusion when pressing a button representing a particular color might have biased the speed of reaction. However, the overall findings prove the validity of the Stroop effect and might be used for further research in cognitive psychology.
Banich, M. T. (2019). The Stroop effect occurs at multiple points along a cascade of control: Evidence from cognitive neuroscience approaches. Frontiers in Psychology, 10, 1-12. Web.
MacLeod, C. M. (1991). Half a century of research on the Stroop effect: An integrative review. Psychological Bulletin, 109 , 163-203.
Megherbi, H., Elbro, C., Oakhill, J., Segui, J., & New, B. (2017). The emergence of automaticity in reading: Effects of orthographic depth and word decoding ability on an adjusted Stroop measure. Journal of Experimental Child Psychology, 166 , 652-663.
Scarpina, F., & Tagini, S. (2017). The Stroop color and word test. Frontiers in Psychology, 8 , 1-8. Web.
Stroop, J. R. (1935). Studies of interference in serial verbal reactions. Journal of Experimental Psychology, 18 , 643-662.
- Chicago (A-D)
- Chicago (N-B)
IvyPanda. (2021, August 8). Experiment in Cognition: Stroop Effect. https://ivypanda.com/essays/experiment-in-cognition-stroop-effect/
"Experiment in Cognition: Stroop Effect." IvyPanda , 8 Aug. 2021, ivypanda.com/essays/experiment-in-cognition-stroop-effect/.
IvyPanda . (2021) 'Experiment in Cognition: Stroop Effect'. 8 August.
IvyPanda . 2021. "Experiment in Cognition: Stroop Effect." August 8, 2021. https://ivypanda.com/essays/experiment-in-cognition-stroop-effect/.
1. IvyPanda . "Experiment in Cognition: Stroop Effect." August 8, 2021. https://ivypanda.com/essays/experiment-in-cognition-stroop-effect/.
Bibliography
IvyPanda . "Experiment in Cognition: Stroop Effect." August 8, 2021. https://ivypanda.com/essays/experiment-in-cognition-stroop-effect/.
- Impact of Age and Sex on Performance of Stroop Activity
- Advanced Addiction Psychology Contemporary Perspectives
- Neuropsychological Diagnosis and Its Main Goals
- Cogmed Working Memory Training in Children
- Human Learning and Non-Human Animal Studies
- Nudges and Human Decision-Making
- Mindfulness as a Practice in Therapy and Daily Life
- Prisoner’s Dilemma in Examples

An official website of the United States government
The .gov means it’s official. Federal government websites often end in .gov or .mil. Before sharing sensitive information, make sure you’re on a federal government site.
The site is secure. The https:// ensures that you are connecting to the official website and that any information you provide is encrypted and transmitted securely.
- Publications
- Account settings
Preview improvements coming to the PMC website in October 2024. Learn More or Try it out now .
- Advanced Search
- Journal List
- Sage Choice
- PMC10798018

The Stroop effect and mental imagery
The classic Stroop task is very simple: you have to name the color of words printed on a page. If these words are color words (like “red” or “blue”), where the color named and the color it is printed in are different (say, “red” printed in blue), the reaction time increases significantly. My aim is to argue that the existing psychological explanations of the Stroop effect need to be supplemented. The Stroop effect is not exclusively about access to motor control. It is also, to a large extent, about interferences in perceptual processing. To put it briefly, reading the color word triggers—laterally and automatically—visual imagery of the color and this interferes with the processing of the perceived color of the word. In other words, the Stroop effect is to a large extent a sensory phenomenon, and it has less to do with attention, conflict monitoring, or other higher-level phenomena.
Introduction
One of the most widely researched psychological phenomena of all times is the Stroop effect (see Stroop, 1935 ). The classic Stroop task is very simple: you have to name the color of words printed on a page. If these words are color words (like “red” or “blue”), where the color named and the color it is printed in are different (say, “red” printed in blue), the reaction time increases significantly.
What explains this odd difference? There are two major explanations, the first one dominant in the second half of the 20th century, the second dominant in the last 20 years. According the first one, the Stroop effect is about attention capture. The linguistic stimulus captures our attention, and as a consequence, less attention remains for the processing of the color stimulus (see MacLeod, 1991 for a summary). According to the second one, the Stroop effect is about conflict monitoring and control: there are control mechanisms that detect the conflict between the linguistic and the color stimulus and they prioritize the processing of the language stimulus ( Botvinick et al., 2001 ). 1
The attention account and the conflict monitoring account of the Stroop effect are very different inasmuch as the former gives a fully bottom-up explanation, whereas the latter a top-down one of the effect the semantic meaning of the word has on the processing of color. But they share an important premise, namely, that the Stroop effect is about access to motor control. Depending on whether the word “red” is printed in red or blue, our access to the motor control (of reading the word) is different and this explains the difference in our reaction time. This is clear enough in the attention account, but it is also what is behind the conflict monitoring account, where “conflict may be operationally defined as the simultaneous activation of incompatible representations […] e.gg., representations of alternative responses” ( Botvinick et al., 2001 , p. 630).
My aim in this paper is to argue that the Stroop effect is not exclusively about access to motor control. It is also, to a large extent, about interferences in perceptual processing. To put it briefly, reading the color word triggers—laterally and automatically—visual imagery of the color and this interferes with the processing of the perceived color of the word.
In section “Mental Imagery”, I outline the concept of mental imagery that is relevant in this discussion and in section “Language Processing and Mental Imagery”, I provide empirical evidence for the various ways in which language processing and mental imagery interact. In section “Back to the Stroop Effect”, I argue that these interactions provide a clear case for a very early perceptual interference from language processing to perceptual processing that explains some aspects of the Stroop effect in a much more straightforward manner than either the attention account or the conflict monitoring account could.
Mental Imagery
The term “mental imagery” was first consistently used in the early days of experimental psychology in the second half of the 19th century and while it has clearly made it to our ordinary language, the way psychologists and neuroscientists use the concept is not as an ordinary language category. Here is a representative definition from a review article on mental imagery in the journal Trends in Cognitive Sciences : “We use the term ‘mental imagery’ to refer to representations […] of sensory information without a direct external stimulus” ( Pearson et al., 2015 , p. 590; see also Nanay, 2015 , 2018 ).
This definition captures the pre-theoretical notion of mental imagery, which we tend to have in mind when, for example, thinking about the experience of closing our eyes and visualizing an apple. That experience is a representation of sensory information without direct external stimulus. But the concept of mental imagery has a much wider scope than just the experience of visualizing.
First, mental imagery, like perception, can happen in all sense modalities. Mental imagery can be visual, but it can also be auditory, olfactory, gustatory, and tactile. Second, while visualizing an apple amounts to a voluntary use of mental imagery, there is also involuntary mental imagery, like flashbacks or earworms—annoying tunes that go through our head in spite of the fact that we really don’t want them to. Third, while in the case of visualizing, mental imagery is not accompanied by the feeling of presence—you’re not actually taking the apple to be in front of you—, some other forms of mental imagery may be accompanied by the feeling of presence, for example, in the case of lucid dreaming and in some forms of hallucinations (which are widely taken to be forms of mental imagery in psychiatry).
The definition I have been using is a negative definition. It defines mental imagery as (to rephrase a bit) sensory representation not triggered directly by sensory input. But it leaves open the question about what this sensory representation is triggered by (directly). In some cases, it is triggered by top-down processes, as in the case of closing your eyes and visualizing an apple. But in other cases, it is triggered laterally, by, for example, input in another sense modality. When you watch the TV muted, for example, your auditory representation (and often your salient auditory experience) is not directly triggered by the auditory input—there is no auditory input as the TV is muted. It is directly triggered by the visual input of the images on TV ( Calvert et al., 1997 ; Hertrich et al., 2011 ; Nanay, 2018 ; Pekkola et al., 2005 ; Spence & Deroy, 2013 ).
It should be clear that while the definition of mental imagery I have been using does seem to capture the ordinary usage of the term, it also carves up mental phenomena somewhat differently. As we have seen, it allows for involuntary imagery. But it also allows for unconscious mental imagery as nothing in the definition says that the perceptual representation that is not triggered directly by sensory input must be a conscious representation.
We have an overwhelming amount of evidence that perception may be conscious or unconscious (e.g., Kouider & Dehaene, 2007 ). But if perceptual representations that are directly triggered by sensory input (i.e., perception) may be unconscious, then it would be arbitrary to posit that perceptual representations that are not directly triggered by sensory input (i.e., mental imagery) may not be. Further, some people report having no conscious mental imagery—these people are called aphantasics and in the last two decades or so a lot of experimental studies were conducted to find out about the causes and nature of aphantasia (see, e.g., Zeman et al., 2007 ). And while aphantasia seems to be a non-monolithic phenomenon, where many different things can lead to the lack of conscious mental imagery, there is clear evidence that at least a subset of aphantasics, while reporting to have no conscious mental imagery at all, do have mental imagery in the sense of perceptual representation that is not directly triggered by sensory input. They have unconscious mental imagery ( Nanay, 2021 ).
In short, mental imagery may be voluntary or involuntary and it may be conscious or unconscious. It is a scientifically respectable (and even publicly observable) category that is well suited to play a role in the explanation of psychological phenomena.
Language Processing and Mental Imagery
We now know that language processing is not completely detachable from mental imagery. Both generating linguistic utterances and hearing/reading them utilizes mental imagery. Some of the empirical findings supporting these claims come from neuroimaging. Describing a scene relies on our ability to generate mental imagery—early cortical representations not directly triggered by sensory input ( Mar, 2004 ; Zadbood et al., 2017 ). Even more importantly, hearing a description invariably triggers mental imagery—again, not necessarily conscious mental imagery, but early cortical representations not directly triggered by sensory input and it is this imagistic representation that is remembered, not the words we heard ( Zwaan, 2016 ; Zwaan & Radvansky, 1998 ).
We understand a fair amount of how this happens and, crucially, we know a lot about the ways in which linguistic labels change (and speed up) perceptual processes and we also know a fair amount about the time scale of this influence. The most important piece of finding both from EEG and from eye tracking studies is that linguistic labels influence shape recognition in less than 100 ms ( Boutonnet & Lupyan, 2015 ; de Groot et al., 2016 ; Noorman et al., 2018 —it should be acknowledged that in these experiments, the onset of the linguistic label preceded the onset of the shape to be recognized). This is a very similar time-frame as how long it takes for the stimulus to reach V4 ( Zamarashkina et al., 2020 )—that is, extremely fast (note that word recognition does take significantly longer, see Hauk et al., 2012 ).
Crucially, this less than 100 ms it takes for linguistic labels to influence shape recognition is much shorter than the time that would be needed for perceptual processing to reach all the way up to higher level representations and then trickle all the way down again to the primary visual cortex (see Lamme & Roelfsema, 2000 ; Thorpe et al., 1996 for the temporal unfolding of visual processing in unimodal cases and see Kringelbach et al., 2015 for a summary of the relative slowness of non-early cortical processing).
By means of comparison, amodal completion (the visual representation of occluded parts of perceived objects) is taken to be bottom-up or laterally influenced on the basis of timing studies although it happens slightly slower than 100 ms. Amodal completion in the early cortices happens within 100–200 ms of retinal stimulation ( Rauschenberger et al., 2001 ; Sekuler & Palmer, 1992 —this is true even of complex visual stimuli, like faces, see Chen et al., 2009 ; see also Lerner et al., 2004 ; Rauschenberger et al. 2006 ; Yun et al., 2018 for detailed studies that track the (very quick) temporal unfolding of amodal completion in different parts of the visual cortex). If the 100–200 ms of amodal completion is explained in terms of lateral influence, then the less than 100 ms of the influence of linguistic labeling can also be explained in terms of lateral influence.
This means that linguistic processing and mental imagery interact at an extremely early stage of perceptual processing—by any account in early cortical processing.
Back to the Stroop Effect
My aim is to argue that in the light of these results about the relation between language processing and mental imagery, we have good reasons to hold that reading the color word triggers—laterally and automatically—visual imagery of the color and this interferes with the processing of the perceived color of the word and this is what explains the Stroop effect. In other words, the conflict between the color and the meaning of the word starts much earlier than motor control.
Here is an experiment that supports this hypothesis directly (there may be some indirect support from findings about the Stroop effect for color-related words as well (like “sky” [for blue] and “fire” [for red]—see Dairymple-Alford, 1972 ). A recent experiment shows that even if we control for all the attentional and other mechanisms that determine motor control, the activation patterns in V4—the part of the visual cortex that is responsible for color processing—would be difficult to explain unless we posit early sensory involvement in the Stroop effect ( Purmann & Pollmann, 2015 ).
Given that V4 is devoted (mainly) to color processing, it is active throughout any color Stroop task. More generally, the involvement of V4 in the Stroop task is somewhat difficult to examine experimentally given that without the functioning of these regions, the effect goes away. So some tricks are required to gain any insight about exactly how early cortical color processing is involved in the Stroop task. The experiments in Purmann & Pollmann, 2015 examined the ways in which the previous trial in a series of Stroop tasks influences the current trial. So the question they raised is how your early sensory cortices behave depending on the order of these trials. If you read the word “red” printed in blue, there is a conflict—it is an “incongruent trial.” If you read the word “blue” printed in blue, there is no conflict—it is referred to as a “congruent trial.”
The question is whether early sensory processing is different depending on whether an incongruent trial was preceded by another incongruent trial. And what the results show is that activities in V4 are very different depending on whether the previous trial was congruent or incongruent. Interestingly, the same effect was not observed in language processing regions of the brain, only in V4. If we take the Stroop task to be about motor control, these results make little sense. But if, as I am suggesting, it is at least partly about sensory processing, these results are exactly what we should expect.
The color of the word activates V4 bottom up (that's perception). And the reading of the word activates V4 laterally and automatically (that's mental imagery). And the processing of the perceived color is slowed down because of the interference of the mental imagery. In short, the conflict between the color and the meaning of the word starts already in perceptual processing.
A word of caution about the scope of the claim I argued for in this paper. While the main findings of the Stroop effect can be explained in terms of the lateral and automatic activation of mental imagery, I don’t want to pretend that all aspects of the Stroop effect can be explained with the help of this explanatory scheme. For example, we know that subjects show greater interference on the first few trials in each block of testing than on subsequent trials in the series ( Henik et al., 1997 ). Also, there is less interference on incongruent trials if they are frequent in comparison with congruent trials ( Lindsey & Jacoby, 1994 ). I don’t think the appeal to the laterally and automatically triggered mental imagery will help us explain these findings.
Nonetheless, we can conclude that the Stroop effect is, at least partially, a sensory phenomenon, and it has less to do with attention, conflict monitoring, or other higher-level phenomena than previously supposed. While it may give us insights into the nature of attention and automaticity or into the intricacies of conflict monitoring and cognitive control, its theoretical import may be even more significant. In fact, the way language processing and perceptual processing interact in the case of the Stroop effect can open up new research directions both about early cortical sensory processing and about language processing, besides touching on some of the deepest (and earliest) philosophical questions about the relation between perception and language.
1. The Stroop effect is an important phenomenon in its own right, but it has also been used in many branches and paradigms of psychological research in order to determine the automaticity of our various psychological responses. Just one example: it is widely held that a necessary feature of synesthetic experiences is that they show the Stroop effect. When deciding whether more unusual forms of synesthesia (e.g., swimming-style synesthesia, where different styles of swimming—backstroke, breaststroke, etc.—bring about synesthetic color experiences, see Nikolić et al., 2011 ; Rothen et al., 2013 ) really counts as genuine synesthesia, the hallmark is to show that they show the Stroop effect.
Author Contribution(s): Bence Nanay: Conceptualization; Formal analysis; Investigation; Project administration; Writing – original draft; Writing – review & editing.
The author declared no potential conflicts of interest with respect to the research, authorship, and/or publication of this article.
Funding: The author received no financial support for the research, authorship, and/or publication of this article.
ORCID iD: Bence Nanay https://orcid.org/0000-0002-2835-6530
Search form (GSE) 1
What the stroop effect reveals about our minds.
The Stroop effect is a simple phenomenon that reveals a lot about how the how the brain processes information.
The Stroop effect is a simple phenomenon that reveals a lot about how the how the brain processes information. First described in the 1930s by psychologist John Ridley Stroop, the Stroop effect is our tendency to experience difficulty naming a physical color when it is used to spell the name of a different color. This simple finding plays a huge role in psychological research and clinical psychology.
The Original Stroop Experiments
In Stroop’s original study, he used three elements: names of colors printed in black ink, names of colors printed in different ink than the color named, and squares of each given color. He then conducted his experiment in two parts:
- In his first experiment, he asked participants to simply read the color printed in black ink. He then asked them to read the words printed, regardless of the color they were printed in.
- For his second experiment, he asked participants to name the ink color instead of the word written. For example, “red” might have been printed in green and participants were asked to identify the color green instead of reading the word “red.” In this segment, participants were also asked to identify the color of the squares.
Stroop found that subjects took longer to complete the task of naming the ink colors of words in experiment two than they took to identify the color of the squares. Subjects also took significantly longer to identify ink colors in experiment two than they had to simply read the printed word in experiment one. He identified this effect as an interference causing a delay in identifying a color when it is incongruent with the word printed.
The Stroop Test
The discovery of the Stroop effect led to the development of the Stroop test. According to an article in Frontiers in Psychology, the Stroop test is used in both experimental and clinical psychology to “assess the ability to inhibit cognitive interference that occurs when processing of a specific stimulus feature impedes the simultaneous processing of a second stimulus attribute.”
In short, the Stroop test, a simplified version of the original experiment, presents incongruent information to subjects by having the color of a word differ from the word printed. The Stroop test can be used to measure a person’s selective attention capacity and skills, processing speed, and alongside other tests to evaluate overall executive processing abilities.
Explanations for the Stroop Effect
A few theories have emerged about why the Stroop effect exists, though there is not widespread agreement about the cause of the phenomenon. Some reasons proposed for the Stroop effect include:
- Selective Attention Theory: According to the second edition of the “Handbook of Psychology,” selective attention chooses “which information will be granted access to further processing and awareness and which will be ignored.” In relation to the Stroop effect, identifying the color of the words takes more attention than simply reading the text. Therefore, this theory suggests that our brains process the written information instead of the colors themselves.
- Automaticity Theory: Our two types of cognitive processing include automatic and controlled thinking. In relation to the Stroop effect, the brain likely reads the word because reading is more of an automated process than recognizing colors.
- Speed of Processing Theory : Simply stated, this theory for the cause of the Stroop effect posits we can process written words faster than we can process colors. Thus, it is difficult to identify the color once we’ve already read the word.
- Parallel Distributed Processing: This theory suggests the brain creates different pathways for different tasks. Therefore, it’s the strength of the pathway that plays an important role in which is easier to name, the color or the text.
Psychologists continue to research the Stroop effect to find the underlying cause for the phenomenon, although many factors have been identified that affect results. For example, some variations in the severity of the Stroop effect are found in women and men. Stroop himself first noted that women experience shorter interruptions than men. Studies have also typically found that older people show longer delays than younger people.
The Impact of the Stroop Effect
It may seem as though the Stroop effect is just a fascinating experiment with no real effect on human psychology. In truth, it illustrates a lot about the way we process information and helps us assess our ability to override our instinctual fast thinking. A study published in the Psychological Review stated, “The effects observed in the Stroop task provide a clear illustration of people’s capacity for selective attention and the ability of some stimuli to escape attentional control.”
The Journal of Experimental Psychology reported that Stroop’s article introducing this phenomenon was among the most cited of the articles they’ve published in their first 100 years. In 2002 as part of its centennial issue , it stated “More than 700 studies have sought to explain some nuance of the Stroop effect; thousands of others have been directly or indirectly influenced by Stroop’s article.”
While the Stroop test is interesting, it also has incredible uses in the world of psychology and the study of the brain. According to a study published on the National Center for Biotechnology Information, the Stroop test is valuable when assessing interference control and task-set coordinating in adults with ADHD . Also, a study published in 1976 found that it was 88.9 percent accurate in distinguishing between clients who had suffered brain damage and those who had not. Later studies confirmed these findings, and the Stroop test is often used to assess selective attention in traumatic brain injury patients .
Multiple studies, including the original experiments by Stroop, suggest that practice can decrease Stroop inference. This has implications for our learning skills, ability to multitask, and how we form habits. Psychologist and economist, Daniel Kahneman explored this concept in his book “Thinking, Fast and Slow.” Our fast thinking, what he refers to as System 1, is our initial, automatic reaction to things we encounter.
Kahneman wrote, “When System 1 runs into difficulty, it calls on System 2 to support more detailed and specific processing that may solve the problem of the moment.” When it comes to the Stroop effect, System 1 (our automatic, fast thinking) seeks to find the quickest pattern available. Kahneman believes by understanding how our brains make connections, we can overcome them to reach more logical conclusions by calling on System 2, our controlled thinking, quicker.
Exploring the Stroop effect continues to play a role in studies and experiments involving automatic and controlled thinking, selective attention, our cognitive processing, and more. Even though the Stroop effect has never been definitively explained, it provides a tried and true benchmark for psychologists and scientists that has been referred to for many years.
Does the study of cognitive processes interest you? Consider an online psychology degree from Lesley University. Our program explores the complexities of the human brain and how it affects behavior. We combine hands-on learning with a robust curriculum, so you’ll be prepared to bring valuable insight to the field of psychology. Plus, our online format allows you the convenience needed to fit your studies into your life.
Related Articles & Stories
Read more about our students, faculty and alumni.
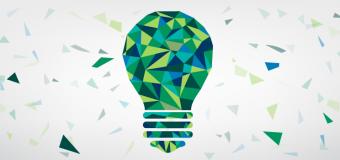
What Can You Do With a Psychology Degree?
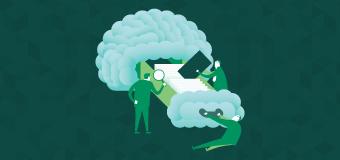
Stages of Memory Formation
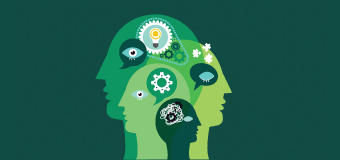
The Science of Personality Development
The Stroop Effect: Insights into Cognitive Interference and Processing Speed
This essay about the Stroop Effect provides a comprehensive exploration of how this psychological phenomenon demonstrates the interaction between attention, reading, and color perception. Named after John Ridley Stroop, the effect occurs when there is a mismatch between the color of the ink and the word itself, leading to errors and slower reaction times. This occurs because of the automatic nature of reading, which competes with the task of color naming. The essay also discusses the broader implications of the Stroop Effect, including its use in diagnosing and understanding attention disorders and its relevance to cognitive neuroscience. Furthermore, it touches on practical applications, such as improving learning environments and understanding multitasking challenges, showcasing the effect’s significance in both academic research and everyday contexts.
How it works
A interesting phenomenon that demonstrates the intricate relationship the human brain has between reading, color perception, and concentration is the Stroop Effect. Since its initial documentation in the 1930s, this effect—named for psychologist John Ridley Stroop—has become a mainstay in psychological research and a fascinating starting point for conversations about cognitive science.
Fundamentally, the Stroop Effect happens when people are asked to identify the color of a word printed in a different hue from the word itself, such as “blue” typed in red ink.
Though the activity appears straightforward, it frequently results in mistakes and slower reaction times. This perplexing result is the result of the colors and words contending for attentional resources, which causes cognitive interference.
Why does this occur? The widely held belief is that for the majority of literate adults, reading is an automatic process. We are compelled to process the semantic meaning of the words as we read literature. Therefore, our brains have to fight the urge to automatically read the word when the job is to state the color of the ink yet the word actually spells out a different color. It’s this tension between word recognition and color identification that causes us to stumble and slow down.
It’s interesting to note that the Stroop Effect illustrates the idea of “automaticity” in cognitive psychology—the notion that certain processes happen without conscious thought. For instance, seasoned drivers are able to converse while driving a familiar route, not even realizing they are driving. Similar to this, reading becomes so automatic that it interferes with the Stroop task without conscious thought.
The Stroop Effect is not only a joke. It has deeper ramifications. It can be used practically to investigate attention disorders like schizophrenia and ADHD. According to studies, people who suffer from these conditions frequently have prominent Stroop interference, which may indicate problems with cognitive control and selective attention. Because of this, researchers are now using the Stroop test as a means of evaluating executive functions and keeping an eye on these disorders.
Furthermore, neuroscientific studies have employed the Stroop Effect to investigate how the brain resolves conflicts among various cognitive tasks. Researchers have found that the anterior cingulate cortex, a region of the brain involved in impulse control and decision-making, is more active during Stroop tests using imaging techniques like as functional magnetic resonance imaging (fMRI). This realization contributes to the understanding of the brain processes behind our capacity to handle conflicting information and prioritize tasks.
Knowing about the Stroop Effect can also help us become more conscious of how multitasking may impact our productivity on a daily basis. Understanding the cognitive burden related to task-switching and interference can help us better manage our time and mental energy in a world where we frequently have to juggle numerous things at once.
Additionally, teachers can help students, especially those with learning difficulties, acquire tactics that decrease cognitive interference by utilizing insights from the Stroop Effect. Teachers can increase learning outcomes and attentiveness by designing learning settings that minimize conflicting inputs.
In summary, the Stroop Effect provides insight into the complex workings of the human mind in addition to being an intriguing psychological phenomenon. It emphasizes the delicate interplay between various cognitive processes and the difficulty of seemingly simple tasks. More understanding of how our brains negotiate the complicated and colorful world around us, as well as improved educational resources and mental health therapies, are all made possible by the progress being made in solving these mysteries.

Cite this page
The Stroop Effect: Insights into Cognitive Interference and Processing Speed. (2024, May 12). Retrieved from https://papersowl.com/examples/the-stroop-effect-insights-into-cognitive-interference-and-processing-speed/
"The Stroop Effect: Insights into Cognitive Interference and Processing Speed." PapersOwl.com , 12 May 2024, https://papersowl.com/examples/the-stroop-effect-insights-into-cognitive-interference-and-processing-speed/
PapersOwl.com. (2024). The Stroop Effect: Insights into Cognitive Interference and Processing Speed . [Online]. Available at: https://papersowl.com/examples/the-stroop-effect-insights-into-cognitive-interference-and-processing-speed/ [Accessed: 21 May. 2024]
"The Stroop Effect: Insights into Cognitive Interference and Processing Speed." PapersOwl.com, May 12, 2024. Accessed May 21, 2024. https://papersowl.com/examples/the-stroop-effect-insights-into-cognitive-interference-and-processing-speed/
"The Stroop Effect: Insights into Cognitive Interference and Processing Speed," PapersOwl.com , 12-May-2024. [Online]. Available: https://papersowl.com/examples/the-stroop-effect-insights-into-cognitive-interference-and-processing-speed/. [Accessed: 21-May-2024]
PapersOwl.com. (2024). The Stroop Effect: Insights into Cognitive Interference and Processing Speed . [Online]. Available at: https://papersowl.com/examples/the-stroop-effect-insights-into-cognitive-interference-and-processing-speed/ [Accessed: 21-May-2024]
Don't let plagiarism ruin your grade
Hire a writer to get a unique paper crafted to your needs.
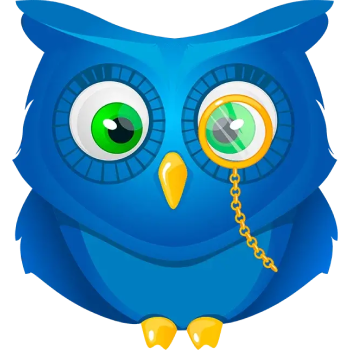
Our writers will help you fix any mistakes and get an A+!
Please check your inbox.
You can order an original essay written according to your instructions.
Trusted by over 1 million students worldwide
1. Tell Us Your Requirements
2. Pick your perfect writer
3. Get Your Paper and Pay
Hi! I'm Amy, your personal assistant!
Don't know where to start? Give me your paper requirements and I connect you to an academic expert.
short deadlines
100% Plagiarism-Free
Certified writers

IMAGES
VIDEO
COMMENTS
PDF | A replication study based on J. Ridley Stroop's original 1935 experiment titled "Studies of Interference in Serial Verbal Reactions". | Find, read and cite all the research you need on ...
The Stroop task is also ubiquitously used in basic and applied research—as indicated by the fact that the original paper (Stroop, 1935) is one of the most cited in the history of ... including the introduction of new ... Focussing on task conflict in the Stroop effect. Psychological Research Psychologische Forschung, 82(2), 284-295. Article ...
The Stroop task is also ubiquitously used in basic and applied research—as indicated by the fact that the original paper (Stroop, 1935) is one of the most cited in the history of psychology and ... including the introduction of new trial ... Half a century of research on the Stroop effect: An integrative review. Psychological Bulletin. ...
Variants of the Classic Stroop Task In essence, Stroop's paradigm provides a template for studying interference,and investigators have often mined that template to create Stroop-like tasks suited to their particular research purposes. Figure 2 illustrates some of the many alternate versions in the literature. The best known is the picture-word
Introduction. The "Stroop effect," named after John Ridley Stroop in the 1930s, is a robust and well-documented (see MacLeod, 1991 for a review) demonstration of interference between two different cognitive processes, namely, an automatic one (e.g., reading) and a controlled one (e.g., naming a color; Stroop, 1935; see also Schneider and Shiffrin, 1977).
One of the most widely researched psychological phenomena of all times is the Stroop effect (see Stroop, 1935 ). The classic Stroop task is very simple: you have to name the color of words printed on a page. If these words are color words (like "red" or "blue"), where the color named and the color it is printed in are different (say ...
One of the most widely researched psychological phenomena of all times is the Stroop effect (see Stroop, 1935). The classic Stroop task is very simple: you have to name the color of words printed on a page. If these words are color words (like "red or blue), where the color named and the color. " " ". it is printed in are different (say ...
The Stroop effect is widely acknowledged as a robust and intriguing behavioral phenomenon referring to a prolonged reaction time when naming the font color of a printed word if this color differs ...
According to a growing consensus, the Stroop effect is understood as a phenomenon of conflict and cognitive control. A tidal wave of recent research alleges that incongruent Stroop stimuli generate conflict, which is then managed and resolved by top-down cognitive control. We argue otherwise: control studies fail to account for major Stroop results obtained over a century-long history of ...
The Stroop task and its corresponding effect are among the best known, most frequently researched phenomena in cognitive psychology (MacLeod 1991; Washburn 2016 ). In its most recognizable form, participants are required to indicate, as quickly as possible, the print color of a series of words, some of which are themselves incompatible color ...
but the "Jaensch-Stroop effect" is an extremely unwieldy label. 3 Stroop changed the color-naming control-condition cards from Ex- periment 2 to Experiment 3. The solid-color squares were replaced by a nonalphanumeric character, which, in Stroop's view, was more like a
This article argues that the Stroop effect can be generated at a variety of stages from stimulus input to response selection. As such, there are multiple loci at which the Stroop effect occurs. Evidence for this viewpoint is provided by a review of neuroimaging studies that were specifically designed to isolate levels of interference in the Stroop task and the underlying neural systems that ...
If this second group showed a Stroop effect only in their second Stroop block, a practice effect must indeed be underlying the hypothesized Group 1 pattern. If however, Group 2 shows the effect already in their first Stroop block, then this difference to the Group 1 pattern must be a consequence of the passage of time, providing an opportunity ...
The Stroop effect is one of the best known phenomena in all of cognitive science and indeed in psychology more broadly. It is also one of the most long standing, having been reported by John Ridley Stroop in the published version of his dissertation in 1935 [1]. In its basic form, the task is to name the color in which a word is printed, ignoring the word itself. When the word is a color word ...
Introduction. The Stroop Color and Word Test (SCWT) is a neuropsychological test extensively used for both experimental and clinical purposes. It assesses the ability to inhibit cognitive interference, which occurs when the processing of a stimulus feature affects the simultaneous processing of another attribute of the same stimulus (Stroop, 1935).In the most common version of the SCWT, which ...
The Stroop effect refers to a delay in reaction times between congruent and incongruent stimuli (MacLeod, 1991). Congruency, or agreement, occurs when a word's meaning and font color are the same. For example, if the word "green" is printed in green. Incongruent stimuli are just the opposite. That is the word's meaning and the color in ...
The EST will show the typical Stroop effect with participants responding quickest to congruent stimuli, then neutral stimuli and slowest to incongruent stimuli 2. There will be an emotional Stroop effect with longer latencies for negative (i.e. sad) stimuli. 3. There will be an age effect with older participants responding faster and more ...
In this research paper, the fundamentals of the Stroop effect, as they are presented by Stroop (1935), will be used as a basis for an experiment investigating the impact of cognitive interference on the time of response to stimuli. The experiment conducted within the realm of this research study is a modification of the original experiment ...
Stroop effect. Naming the displayed color of a printed word is an easier and quicker task if the word matches the color (top) than if it does not (bottom). In psychology, the Stroop effect is the delay in reaction time between congruent and incongruent stimuli. The effect has been used to create a psychological test (the Stroop test) that is ...
The Stroop effect is an important phenomenon in its own right, but it has also been used in many branches and paradigms of psychological research in order to determine the automaticity of our various psychological responses. Just one example: it is widely held that a necessary feature of synesthetic experiences is that they show the Stroop effect.
I. INTRODUCTION If one is seeking out a relatively simple and engaging experiment to examine how the brain recognizes images while being distracted, replicating the Stroop effect can provide interesting results. The famous "Stroop Effect" is named after J. Ridley Stroop who discovered this phenomenon in the 1930s. The
Stroop Effect Research Paper Introduction - Free download as PDF File (.pdf), Text File (.txt) or read online for free. The document discusses the challenges of writing a thesis on the Stroop effect, a cognitive phenomenon where it takes longer to name the color of the ink that a color word is printed in when the word and color are incongruent.
The Stroop effect is a simple phenomenon that reveals a lot about how the how the brain processes information. First described in the 1930s by psychologist John Ridley Stroop, the Stroop effect is our tendency to experience difficulty naming a physical color when it is used to spell the name of a different color. This simple finding plays a huge role in psychological research and clinical ...
This essay about the Stroop Effect provides a comprehensive exploration of how this psychological phenomenon demonstrates the interaction between attention, reading, and color perception. Named after John Ridley Stroop, the effect occurs when there is a mismatch between the color of the ink and the word itself, leading to errors and slower ...