Thank you for visiting nature.com. You are using a browser version with limited support for CSS. To obtain the best experience, we recommend you use a more up to date browser (or turn off compatibility mode in Internet Explorer). In the meantime, to ensure continued support, we are displaying the site without styles and JavaScript.
- View all journals
- Explore content
- About the journal
- Publish with us
- Sign up for alerts
- Published: 01 February 2006
The Blue Brain Project
- Henry Markram 1
Nature Reviews Neuroscience volume 7 , pages 153–160 ( 2006 ) Cite this article
12k Accesses
763 Citations
168 Altmetric
Metrics details
IBM's Blue Gene supercomputer allows a quantum leap in the level of detail at which the brain can be modelled. I argue that the time is right to begin assimilating the wealth of data that has been accumulated over the past century and start building biologically accurate models of the brain from first principles to aid our understanding of brain function and dysfunction.
This is a preview of subscription content, access via your institution

Access options
Subscribe to this journal
Receive 12 print issues and online access
176,64 € per year
only 14,72 € per issue
Buy this article
- Purchase on Springer Link
- Instant access to full article PDF
Prices may be subject to local taxes which are calculated during checkout
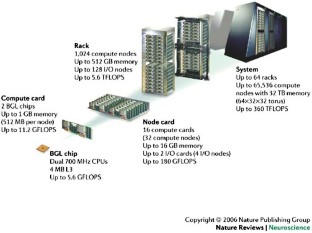
Similar content being viewed by others
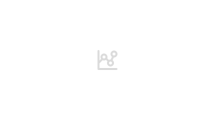
A body–brain circuit that regulates body inflammatory responses
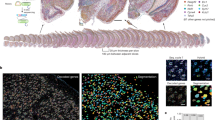
Whole-cortex in situ sequencing reveals input-dependent area identity
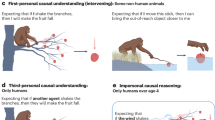
The development of human causal learning and reasoning
The Blue Brain Project [online], < http://bluebrainproject.epfl.ch > (2005).
Blue Gene [online], < http://www.research.ibm.com/bluegene > (2005).
Deep Blue [online], < http://www.research.ibm.com/deepblue > (2005).
Hodgkin, A. L. & Huxley, A. F. A quantitative description of membrane current and its application to conduction and excitation in nerve. J. Physiol. (Lond.) 117 , 500–544 (1952).
CAS Google Scholar
Rall, W. Branching dendritic trees and motoneuron membrane resistivity. Exp. Neurol. 1 , 491–527 (1959).
Article CAS Google Scholar
Segev, I. & Rall, W. Excitable dendrites and spines: earlier theoretical insights elucidate recent direct observations. Trends Neurosci. 21 , 453–460 (1998).
Johnston, D. et al. Active dendrites, potassium channels and synaptic plasticity. Phil. Trans. R. Soc. Lond. B 358 , 667–674 (2003).
Magee, J. C. Dendritic integration of excitatory synaptic input. Nature Rev. Neurosci. 1 , 181–190 (2000).
London, M. & Hausser, M. Dendritic computation. Annu. Rev. Neurosci. 28 , 503–532 (2005).
Migliore, M. & Shepherd, G. M. Emerging rules for the distributions of active dendritic conductances. Nature Rev. Neurosci. 3 , 362–370 (2002).
Rall, W. & Shepherd, G. M. Theoretical reconstruction of field potentials and dendrodendritic synaptic interactions in olfactory bulb. J. Neurophysiol. 31 , 884–915 (1968).
Pellionisz, A., Llinas, R. & Perkel, D. H. A computer model of the cerebellar cortex of the frog. Neuroscience 2 , 19–35 (1977).
Shepherd, G. M. & Brayton, R. K. Computer simulation of a dendrodendritic synaptic circuit for self- and lateral-inhibition in the olfactory bulb. Brain Res. 175 , 377–382 (1979).
Traub, R. D. & Wong, R. K. Cellular mechanism of neuronal synchronization in epilepsy. Science 216 , 745–747 (1982).
Traub, R. D., Miles, R. & Buzsaki, G. Computer simulation of carbachol-driven rhythmic population oscillations in the CA3 region of the in vitro rat hippocampus. J. Physiol. (Lond.) 451 , 653–672 (1992).
Douglas, R. J. & Martin, K. A. C. A functional microcircuit for cat visual cortex. J. Physiol. (Lond.) 440 , 735–769 (1991).
Wang, X. J. & Rinzel, J. Spindle rhythmicity in the reticularis thalami nucleus: synchronization among mutually inhibitory neurons. Neuroscience 53 , 899–904 (1993).
De Schutter, E. & Bower, J. M. An active membrane model of the cerebellar Purkinje cell. I. Simulation of current clamps in slice. J. Neurophysiol. 71 , 375–400 (1994).
Bush, P. & Sejnowski, T. J. Inhibition synchronizes sparsely connected cortical neurons within and between columns in realistic network models. J. Comput. Neurosci. 3 , 91–110 (1996).
Contreras, D., Destexhe, A., Sejnowski, T. J. & Steriade, M. Control of spatiotemporal coherence of a thalamic oscillation by corticothalamic feedback. Science 274 , 771–774 (1996).
Destexhe, A., Bal, T., McCormick, D. A. & Sejnowski, T. J. Ionic mechanisms underlying synchronized oscillations and propagating waves in a model of ferret thalamic slices. J. Neurophysiol. 76 , 2049–2070 (1996).
Golomb, D. & Amitai, Y. Propagating neuronal discharges in neocortical slices: computational and experimental study. J. Neurophysiol. 78 , 1199–1211 (1997).
Lytton, W. W., Contreras, D., Destexhe, A. & Steriade, M. Dynamic interactions determine partial thalamic quiescence in a computer network model of spike-and-wave seizures. J. Neurophysiol. 77 , 1679–1696 (1997).
Destexhe, A., Contreras, D. & Steriade, M. Cortically-induced coherence of a thalamic-generated oscillation. Neuroscience 92 , 427–443 (1999).
Egger, V., Feldmeyer, D. & Sakmann, B. Coincidence detection and changes of synaptic efficacy in spiny stellate neurons in rat barrel cortex. Nature Neurosci. 2 , 1098–1105 (1999).
Bal, T., Debay, D. & Destexhe, A. Cortical feedback controls the frequency and synchrony of oscillations in the visual thalamus. J. Neurosci. 20 , 7478–7488 (2000).
Howell, F. W., Dyrhfjeld-Johnsen, J., Maex, R., Goddard, N. & De Schutter, E. A large scale model of the cerebellar cortex using PGENESIS. Neurocomputing 32 , 1036–1041 (2000).
Google Scholar
Kozlov, A., Kotaleski, J. H., Aurell, E., Grillner, S. & Lansner, A. Modeling of substance P and 5-HT induced synaptic plasticity in the lamprey spinal CPG: consequences for network pattern generation. J. Comput. Neurosci. 11 , 183–200 (2001).
Bazhenov, M., Timofeev, I., Steriade, M. & Sejnowski, T. J. Model of thalamocortical slow-wave sleep oscillations and transitions to activated states. J. Neurosci. 22 , 8691–8704 (2002).
Pinto, D. J., Jones, S. R., Kaper, T. J. & Kopell, N. Analysis of state-dependent transitions in frequency and long-distance coordination in a model oscillatory cortical circuit. J. Comput. Neurosci. 15 , 283–298 (2003).
Article Google Scholar
Bazhenov, M., Timofeev, I., Steriade, M. & Sejnowski, T. J. Potassium model for slow (2–3 Hz) in vivo neocortical paroxysmal oscillations. J. Neurophysiol. 92 , 1116–1132 (2004).
Traub, R. D. et al. Single-column thalamocortical network model exhibiting gamma oscillations, sleep spindles, and epileptogenic bursts. J. Neurophysiol. 93 , 2194–2232 (2005).
Thomson, A. M., Girdlestone, D. & West, D. C. Voltage-dependent currents prolong single-axon postsynaptic potentials in layer III pyramidal neurons in rat neocortical slices. J. Neurophysiol. 60 , 1896–1907 (1988).
Dodt, H. U. & Zieglgansberger, W. Visualizing unstained neurons in living brain slices by infrared DIC-videomicroscopy. Brain Res. 537 , 333–336 (1990).
Stuart, G. J., Dodt, H. U. & Sakmann, B. Patch-clamp recordings from the soma and dendrites of neurons in brain slices using infrared video microscopy. Pflugers Arch. 423 , 511–518 (1993).
Markram, H. et al. Interneurons of the neocortical inhibitory system. Nature Rev. Neurosci. 5 , 793–807 (2004).
Martin, K. A. Microcircuits in visual cortex. Curr. Opin. Neurobiol. 12 , 418–425 (2002).
Silberberg, G., Gupta, A. & Markram, H. Stereotypy in neocortical microcircuits. Trends Neurosci. 25 , 227–230 (2002).
Toledo-Rodriguez, M. et al. Correlation maps allow neuronal electrical properties to be predicted from single-cell gene expression profiles in rat neocortex. Cereb. Cortex 14 , 1310–1327 (2004).
NEURON [online], < http://www.neuron.yale.edu/neuron > (2005).
Tsodyks, M., Pawleslik, K. & Markram, H. Neural networks with dynamic synapses. Neural Comput. 10 , 821–835 (1998).
NeoCortical Simulator [online], < http://brain.cse.unr.edu/ncsDocs > (2005).
SGI [online], < http://www.SGI.com > (2005).
The Human Brain Project [online], < http://www.nimh.nih.gov/neuroinformatics > (2005).
Markram, H. Dendritic object theory: a theory of the neural code where 3D electrical objects are formed across dendrites by neural microcircuits. Swiss Soc. Neurosci. Abstr. 196 (2005).
Download references
Acknowledgements
I am grateful for the efforts of all my students, especially Y. Wang, A. Gupta, M. Toledo and G. Silberberg, in carrying out such challenging experiments and producing such incredible data. I thank P. Aebischer, G. Margaritondo, F. Avellan, G. Parisod and the entire EPFL (Ecole Polytechnique Fédérale de Lausanne) administration for their support of this project and for acquiring Blue Gene. I thank IBM (International Business Machines) for making this prototype supercomputer available and for their major support of neuroscience. I also thank SGI (Silicon Graphics, Inc.) for their major initiative to help with the visualization of the Blue Brain. I thank P. Goodman for his long-standing support of our reconstruction efforts and for introducing me to the Blue Gene initiative in 2000. Thanks also to the US Office of Naval Research for their support. I thank I. Segev, who is and will be essential to the success of the project, and G. Shepherd for their valuable comments on the manuscript.
Author information
Authors and affiliations.
the Laboratory of Neural Microcircuitry, Brain Mind Institute, Ecole Polytechnique Fédérale de Lausanne, Lausanne, 1015, Switzerland
Henry Markram
You can also search for this author in PubMed Google Scholar
Ethics declarations
Competing interests.
The author declares no competing financial interests.
Related links
Further information, rights and permissions.
Reprints and permissions
About this article
Cite this article.
Markram, H. The Blue Brain Project. Nat Rev Neurosci 7 , 153–160 (2006). https://doi.org/10.1038/nrn1848
Download citation
Issue Date : 01 February 2006
DOI : https://doi.org/10.1038/nrn1848
Share this article
Anyone you share the following link with will be able to read this content:
Sorry, a shareable link is not currently available for this article.
Provided by the Springer Nature SharedIt content-sharing initiative
This article is cited by
Multiple neuron clusters on micro-electrode arrays as an in vitro model of brain network.
- Martina Brofiga
- Serena Losacco
- Bruno Burlando
Scientific Reports (2023)
Uncertainty quantification and sensitivity analysis of a hippocampal CA3 pyramidal neuron model under electromagnetic induction
- Muhammad Bilal Ghori
- Yanmei Kang
Nonlinear Dynamics (2023)
Petabyte-Scale Multi-Morphometry of Single Neurons for Whole Brains
- Shengdian Jiang
- Hanchuan Peng
Neuroinformatics (2022)
The Neuron Phenotype Ontology: A FAIR Approach to Proposing and Classifying Neuronal Types
- Thomas H. Gillespie
- Shreejoy J. Tripathy
- Sean L. Hill
Quick links
- Explore articles by subject
- Guide to authors
- Editorial policies
Sign up for the Nature Briefing newsletter — what matters in science, free to your inbox daily.

Academia.edu no longer supports Internet Explorer.
To browse Academia.edu and the wider internet faster and more securely, please take a few seconds to upgrade your browser .
Enter the email address you signed up with and we'll email you a reset link.
- We're Hiring!
- Help Center
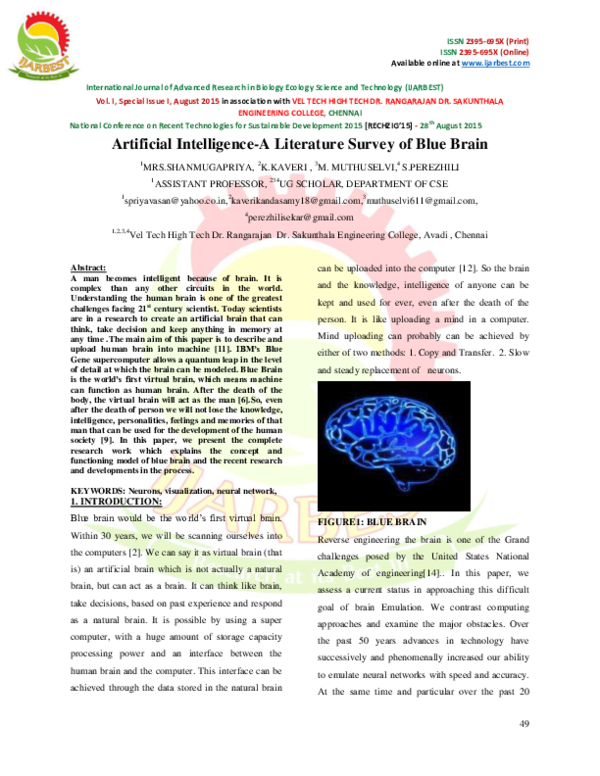
Artificial Intelligence-A Literature Survey of Blue Brain.pdf

Related Papers
IJESRT Journal
The Blue Brain Project is the first made comprehensive attempt to reverse-engineer the brain of mammalian, so that through detailed simulations the function of brain can be understood. BLUE BRAIN is the name of the world's first virtual brain which means, a machine that can function as human brain. Today, scientists are in research to create an artificial brain that can think, respond, take decision, and store anything in memory. The main aim of this research is to upload human brain into machine. So that man can think and take decision without any effort. After the death of the body, the virtual brain will act as the man. So, even after the death of a person we will not lose the knowledge, intelligence, personalities, feelings and memories of that man that can be used for the development of the human society. In this paper, we present the complete research work which explains the concept and functioning model of blue brain and the recent research and developments in the process.
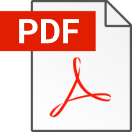
International Journal for Research in Applied Science and Engineering Technology
Uday Patkar
Journal of emerging technologies and innovative research
Blue brain, world’s first virtual brain. It is coined as a machine that function as a human brain. In the present scenario scientists and researchers are in research to create an artificial brain that can think, response, take decision and keep anything in memory. The main concept of blue brain is to upload human brain into machine, So that man can think, take decision without any effort. The fact is that after the death of the body, the virtual brain will be acted as man. So after the death of a person, world will not lose the knowledge, intelligence, personalities, feelings and memories of that man that can be used for the development of the human society. This study has made an attempt to review critically review the concept of Blue Brain. Index Terms Blue Brain, artificial brain, Nanobots
Journal of Informatics Electrical and Electronics Engineering (JIEEE), A2Z Journals
Journal of Informatics Electrical and Electronics Engineering (JIEEE), A 2 Z Journals
Blue brain is a supercomputer programmed such that it can function as an artificial brain, which can also be called a virtual brain. IBM is developing this virtual brain which would be the world’s first such created machine. Its main aim is to create a machine in which the information of the actual brain can be uploaded. This would ensure that a person’s knowledge, personality, memories, and intelligence are preserved and safe. The Blue Brain project utilizes the technologies of reverse engineering and artificial intelligence at its core and is implemented through the use of supercomputers and nanobots. Special software like BBP-SDK are also specifically developed for the Blue Brain project. The Blue Brain project is centered towards finding viable solutions to brain-disorders, a working model close to the actual brain which would help in greater understanding of the human brain and the human mind and the state of consciousness, a step towards building an independently thinking machine, and finally collecting information of hundreds of years from the human brains and storing it in the form of a databases. The Blue Brain project mimics the human brain by acquiring the data from its surrounding through special software, interpreting through neural electrophysiology and morphology, and simulating them on computers. Thus, The Blue Brain project is a powerful tool for the study and analysis of the human brain and for the advancement of the human brain and society
International Journal for Research in Applied Science & Engineering Technology (IJRASET)
IJRASET Publication
Blue brain" is the name of the world's first virtual brain. It allows to transfer all the substances of human brain to virtual brain like PC. That means a machine can function as human brain. In other words, human is does not live for thousands of years but the information in his mind could be saved and used for several thousands of years. Today scientists are in research to create an artificial brain that can think, response, take decision, and keep anything in memory. The main aim is to upload human brain into machine. So that man can think, take decision without any effort. After the death of the person the virtual brain can store the knowledge, intelligence, personalities, feelings and memories of that person that can be used for the development of the human society.
IJSTE - International Journal of Science Technology and Engineering
Blue brain is a Virtual Brain it is the creation of synthetic brain by reverse engineering and recreates it at the cellular level inside a computer simulation. The concept of blue brain founded in May 2005 by Henry Markram at the EPFL in Lausanne, Switzerland. The aim of the project to gain a complete understanding of the brain and to enable better and faster development of brain disease treatments. No one can ever understand the complexity of human brain. It is more complex than any circuitry in the world. The scientists today are in research to create an artificial brain that can think, respond, take decision, and keep everything in memory. After the death of the body, we will not lose knowledge, intelligence, feelings and memory of that man and can be used for the welfare of human society.
Bala Sriram Kodi
—Human brain is the invaluable and quintessential creation of god, but search creation comes to end when a person reaches to the last phase of his life. The man acquires the knowledge and responds to the things because of the brain. In this research paper I used to describe how a human brain intelligence can be preserve for the future generation and how the Man-Machine-Man (M 3) interface is possible with the blue brain project. The main aim of this project is to upload human brain into machine. So, that man can think, take decision without any effort. After the death of the body, the virtual brain will act's as the man. So even after the death of a person we will not lose the knowledge, intelligence, personalities, feelings and memories of the man. That can be used for the development of the human society. This paper has a information about the stages, requirements of the modules to interface, merits and demerits of the technology for the human world.
Prof. Dr. SASIDHAR BABU SUVANAM
Human brain, the greatest creation of god which is package of unimaginative functions. A man is intelligent because of the brain. " Blue Brain " is world's first virtual brain. Pink brain is special because of that it can think, respond, take decision without any effort and keep anything in memory. Aim of the project is to archive features of Pink brain to a Digital system. In short, " Brain to a Digital System ". After death of a human, data including intelligence, knowledge, personality, memory and feelings can be used for further development of society. BB Storage space is an extracted concept from Blue brain project. Storing numerous and variety of data on memory is an advantage provided. By this concept, registers act as neurons and Electric signals as simulation impulses. Variation of data are identified based on signal variation that reach the registers. Registers mentioned here is same that a normal system maintains. Benefit 2126 Y.Vijayalakshmi et al focused by this concept of storage is storing data without deletion on real time as normal brain does. Attaching this concept to an expert system reflects drastic changes while it responds. To make the system more friendly a regional Language conversion using Natural Language Processing (NLP) is included with a voice recognition technique. In similar way, system should also respond using the experiences that it has been traveled through, in voice format. This is the output format of BB storage space. Nanobots on Blue brain which is used for information collection from neurons is replaced by real time experiences provided to the machine.
Ashutosh Verma
Saurav Poonia
We all are fascinated about how human brain works and why it is so superior and extraordinary. As some of the brains (Einstein, Ford, Edison and so on) in world are too resourceful and astonishing were also had the similar human brain but functioning is differ. To understand those workings, we require Blue brain. This technology is somewhere more state-of-the-art and groundbreaking than others. Even after the death of an individual, his/her brain will be alive virtually. In this paper an overview, modeling and recommendations for Blue brain has been presented for conceptual information. Through study it is also found that there are some challenges faced by this revolutionary technology. The simulation of human or mammalian brain with Blue brain is to identify the fundamental principles of brain structure and function in health and disease.
Blue Brain - The Magic of Man
Ieee account.
- Change Username/Password
- Update Address
Purchase Details
- Payment Options
- Order History
- View Purchased Documents
Profile Information
- Communications Preferences
- Profession and Education
- Technical Interests
- US & Canada: +1 800 678 4333
- Worldwide: +1 732 981 0060
- Contact & Support
- About IEEE Xplore
- Accessibility
- Terms of Use
- Nondiscrimination Policy
- Privacy & Opting Out of Cookies
A not-for-profit organization, IEEE is the world's largest technical professional organization dedicated to advancing technology for the benefit of humanity. © Copyright 2024 IEEE - All rights reserved. Use of this web site signifies your agreement to the terms and conditions.
Blue Brain Technology
- First Online: 04 March 2020
Cite this chapter
- Akshay Tyagi 13 &
- Laxmi Ahuja 13
Part of the book series: Lecture Notes in Networks and Systems ((LNNS,volume 103))
918 Accesses
After death, the human body gets destroyed, brain stops working and human eventually loses his/her knowledge of the brain. But this knowledge and information can be preserved and used for thousands of years. Blue brain is the name of the first virtual brain in the world. This technology helps this activity. This article contains information about the blue brain, its needs, blue brain-building strategies, strengths and weaknesses and more. Collect data on the many types of somatic cells. The analog squares measurements were published on a IBM blue-chip central computer, hence the name “Blue Brain.” This usually corresponds to the size of the bee’s brain. It is hoped that simulation of gallium in the rat brain (21 million neurons) is to be performed by 2014. If you receive enough money, a full simulation of the human brain (86 billion neurons) should be performed, here 2023.
This is a preview of subscription content, log in via an institution to check access.
Access this chapter
- Available as EPUB and PDF
- Read on any device
- Instant download
- Own it forever
- Compact, lightweight edition
- Dispatched in 3 to 5 business days
- Free shipping worldwide - see info
- Durable hardcover edition
Tax calculation will be finalised at checkout
Purchases are for personal use only
Institutional subscriptions
de Camargo RY (2011) A multi-GPU algorithm for communication in neuronal network simulations. In: 2011 18th International conference on high performance computing (HiPC), pp 1–10
Google Scholar
Sandberg A, Boström N (2008). Whole brain emulation: a roadmap
Johanson C, Lansner A, Towards cortex estimated fake neural frameworks. Neural Netw
https://www.theglobaljournals.com/ijar/val=August1374511934_1c167_50.pdf
https://archive.org/stream/SingularityWikibook/Singularity-Wikibook_djvu.txt
Farahini N, Hemani A (2013) A conceptual custom super-computer design for real-time simulation of human brain. In: 2013 21st Iranian conference on electrical engineering (ICEE), pp 1–6
Painkras E, Plana LA, Garside J, Temple S, Galluppi F, Patterson C, Lester DR, Brown AD, Furber SB (2013) SpiNNaker: a 1-W 18-core system-on-chip for massively-parallel neural network simulation. Solid-State Circuits IEEE J 48(8):1943–1953
Article Google Scholar
Preissl R, Pallab T, Flikner M, Singh R, Esser SK, Risk W, Simon H, Modha DS Compass: a scalable simulator for an architecture for cognitive computing
Wang M, Yan B, Hu J, Li P (2011) Simulation of large neuronal networks with biophysically accurate models on graphics processors. In: The 2011 international joint conference on neural networks (IJCNN), pp 3184–3193
Claton K, Rae B, Nancy, Charbn E, Hendeson RK, Leng G, Murray A (2011) An implementation of a spike-response model with escape noise using an avalanche diode. Biomed Circuits Syst IEEE Trans 5(3):231–243
Download references
Acknowledgements
The authors express their deep sense of gratitude to the founding President of Amity University, Mr. Ashok K. Chauhan, for his great interest in promoting research at Amity University and for his motivation to reach new heights.
Author information
Authors and affiliations.
Amity Institute of Information Technology, Amity University, Noida, Uttar Pradesh, India
Akshay Tyagi & Laxmi Ahuja
You can also search for this author in PubMed Google Scholar
Editor information
Editors and affiliations.
Guru Nanak Institutions, Hyderabad, Telangana, India
Harvinder Singh Saini
Rishi Sayal
School of Computing and Information Systems, The University of Melbourne, Melbourne, VIC, Australia
Rajkumar Buyya
Department of Computer Science and Engineering, Jawaharlal Nehru Technological University Hyderabad, Hyderabad, Telangana, India
Govardhan Aliseri
Rights and permissions
Reprints and permissions
Copyright information
© 2020 Springer Nature Singapore Pte Ltd.
About this chapter
Tyagi, A., Ahuja, L. (2020). Blue Brain Technology. In: Saini, H., Sayal, R., Buyya, R., Aliseri, G. (eds) Innovations in Computer Science and Engineering. Lecture Notes in Networks and Systems, vol 103. Springer, Singapore. https://doi.org/10.1007/978-981-15-2043-3_11
Download citation
DOI : https://doi.org/10.1007/978-981-15-2043-3_11
Published : 04 March 2020
Publisher Name : Springer, Singapore
Print ISBN : 978-981-15-2042-6
Online ISBN : 978-981-15-2043-3
eBook Packages : Engineering Engineering (R0)
Share this chapter
Anyone you share the following link with will be able to read this content:
Sorry, a shareable link is not currently available for this article.
Provided by the Springer Nature SharedIt content-sharing initiative
- Publish with us
Policies and ethics
- Find a journal
- Track your research
- Open access
- Published: 27 April 2024

Obesity-induced blood-brain barrier dysfunction: phenotypes and mechanisms
- Ziying Feng 1 , 2 ,
- Cheng Fang 1 ,
- Yinzhong Ma 1 , 3 &
- Junlei Chang 1 , 2 , 3
Journal of Neuroinflammation volume 21 , Article number: 110 ( 2024 ) Cite this article
770 Accesses
18 Altmetric
Metrics details
Obesity, a burgeoning global health issue, is increasingly recognized for its detrimental effects on the central nervous system, particularly concerning the integrity of the blood-brain barrier (BBB). This manuscript delves into the intricate relationship between obesity and BBB dysfunction, elucidating the underlying phenotypes and molecular mechanisms. We commence with an overview of the BBB’s critical role in maintaining cerebral homeostasis and the pathological alterations induced by obesity. By employing a comprehensive literature review, we examine the structural and functional modifications of the BBB in the context of obesity, including increased permeability, altered transport mechanisms, and inflammatory responses. The manuscript highlights how obesity-induced systemic inflammation and metabolic dysregulation contribute to BBB disruption, thereby predisposing individuals to various neurological disorders. We further explore the potential pathways, such as oxidative stress and endothelial cell dysfunction, that mediate these changes. Our discussion culminates in the summary of current findings and the identification of knowledge gaps, paving the way for future research directions. This review underscores the significance of understanding BBB dysfunction in obesity, not only for its implications in neurodegenerative diseases but also for developing targeted therapeutic strategies to mitigate these effects.
Introduction
Obesity, characterized by excessive fat accumulation, represents a burgeoning health issue of global populations. The World Health Organization reports an alarming rise in obesity rates worldwide, with an estimated 2.5 billion adults being overweight (body mass index, BMI > 25), of which approximately 890 million are obese (BMI > 30) as of 2022 [ 1 ]. This pervasive phenomenon profoundly impacts human health, inciting a multitude of disorders including cardiovascular disease, stroke, diabetes, and certain types of cancer.
Of particular interest, however, is the intricate relationship between obesity and neurological disorders, a link that has increasingly captivated scientific attention. Alzheimer’s disease [ 2 , 3 , 4 ] and stroke [ 5 , 6 , 7 ], for instance, are two representative neurological disorders that have been recurrently associated with obesity. Recent evidence points to obesity as a critical risk factor for mitochondrial dysfunction, as astrocytic fatty acid oxidation (FAO) and oxidative phosphorylation (OxPhos) are involved in these lipid-involving neurodegenerative disorders, implicating obesity’s role in influencing the disease onset and progression [ 8 , 9 ]. This escalating prevalence of obesity and its potential implications for neurological disorders presents a grave concern for global health. It necessitates a comprehensive understanding of obesity’s detrimental consequences towards the central nervous system (CNS), specifically its effects on the cerebral vascular system.
A critical component of the cerebrovascular system is the blood-brain barrier (BBB), a critical function of brain blood microvessels that maintains CNS homeostasis. The BBB strictly regulates the exchange of substances between the blood and the brain. This dynamic barrier ensures the stability of brain microenvironment, protects against neurotoxins, and facilitates proper neuronal function [ 10 , 11 ]. However, in pathological states such as obesity, this pivotal barrier can be compromised, leading to various neurological disorders. Understanding the intricate relationship between obesity and BBB dysfunction therefore becomes a matter of paramount importance.
We aim to delve into the complexities of this relationship, exploring the phenotypes and underlying mechanisms of BBB dysfunction in obesity. Through a comprehensive examination of the current literature, we provide an in-depth analysis of the potential changes in BBB structure and function induced by obesity. Additionally, we elucidate the mechanistic pathways that may be implicated in BBB dysfunction in obesity. This review ultimately seeks to underscore the significance of understanding BBB dysfunction in obesity and its implications for neurological health, with the goal of guiding future research and clinical practice.
Blood-brain barrier: cellular and molecular components
The blood-brain barrier (BBB) is a sophisticated and highly specialized biological construct, known for its selective permeability and protective role within the CNS. It owes its unique functionality to its intricately assembled cellular and molecular components, which work together to maintain CNS homeostasis. The structural and functional foundation of the BBB is the neurovascular unit (NVU) [ 12 ], a complex ensemble encompassing brain endothelial cells (BECs), pericytes, astrocytes, extracellular matrix (ECM), microglia, and neurons (Fig. 1 ).
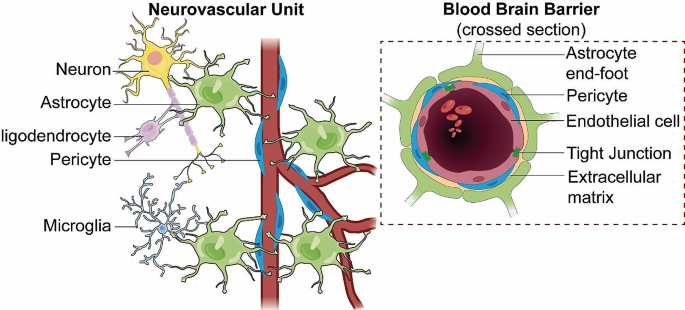
BBB integrated within the NVU . This diagram depicts the structural components of the blood-brain barrier (BBB) within the neurovascular unit (NVU), illustrating the tight junctions of endothelial cells, the supportive pericytes, and the encircling astrocytic end-feet. It highlights the BBB’s integral structural role in the NVU, which is essential for maintaining cerebral integrity and function
Endothelial cells
As the primary component of the BBB, brain endothelial cells (BECs), line the interior surfaces of the brain’s capillaries. These cells are distinctive in their unique morphology and possess attributes that separate them from peripheral ECs [ 13 , 14 , 15 ]. BECs are closely interconnected through tight junctions (TJs), which restrict the paracellular pathway and control the ionic balance across the barrier. This feature is instrumental in preventing the free passage of substances from the blood into the brain. BECs also exhibit limited pinocytic activity and lack fenestrations, thereby further restricting transcellular transport. In order to provide brain with abundant nutrients and a healthy environment, specific transporters which allows for the selective passage of molecules are expressed in BECs. Through analyzing single-cell RNA-sequencing data of mouse ECs from different organs provided by the Tabula Muris consortium, Paik et al. found that BECs possess not only its own unique transcriptomic identities, but also the most specialized differentially expressed genes (DEGs) profiles that expressed primarily solute carrier transporters [ 16 ]. For instance, glucose transporter protein-1(Glut-1) is responsible for transporting glucose, which being a crucial energy fuel, from blood to the brain [ 17 ]. On the other hand, P-glycoprotein (P-gp) acts as an efflux pump that removes harmful substances out of the brain to keep a safe environment [ 18 ].
Worth noticed, BECs can also transport molecules through low rates of transcytosis, a process that plays a vital role in endothelial transport [ 19 , 20 ]. Transcytosis allows for selective or non-selective passage of molecules across the endothelial cells. In BECs, transcytosis can be divided into receptor-mediated (selective) transcytosis, which includes the transport of transferrin and insulin, and bulk-phase or fluid-phase (non-selective) transcytosis, typically exemplified by the transport of albumin [ 21 ]. Notably, while native albumin crosses the BBB via non-selective bulk-phase transcytosis, cationic albumin is transported more efficiently as it engages in adsorptive transcytosis, highlighting the distinct pathways for different forms of albumin. A key regulator in this process is Major Facilitator Superfamily Domain Containing 2 A (Mfsd2a), which specifically inhibits bulk-phase transcytosis in BECs [ 22 ]. Mfsd2a modifies the lipid composition of the cell membrane, thereby limiting the formation of caveolae vesicles and reducing caveolae-mediated transcytosis. This action helps maintain the selective permeability and overall integrity of the BBB. Contrary to inhibiting all forms of transcytosis, Mfsd2a’s role is particularly pivotal in restraining non-selective transcytosis or bulk-phase transcytosis. Research indicates that overexpression of Mfsd2a in CNS endothelial cells leads to a decreased number of transcytotic vesicles, which in turn reduces hematoma levels and alleviates BBB injury in mouse models of intracerebral hemorrhage (ICH) [ 23 ]. This highlights the potential therapeutic value of Mfsd2a in mitigating BBB disruption by specifically targeting non-selective transcytosis mechanisms post-injury.
In comparison to endothelial cells found elsewhere in the body, brain endothelial cells (BECs) demonstrate a unique profile of leukocyte adhesion molecule (LAM) expression. This profile contributes to the CNS’s immune privilege by limiting the extravasation of leukocytes under normal conditions. While LAMs are integral to leukocyte adhesion and migration — key steps in the immune response — their regulated expression in BECs ensures a controlled interaction with leukocytes, thereby maintaining CNS homeostasis [ 24 ]. Contrary to the constant expression in peripheral tissues, the presence and activity of LAMs in the BBB vary contextually. Notably, BECs typically lack selectins in their Weibel-Palade bodies, which reduces their ability for rapid leukocyte recruitment compared to peripheral endothelial cells. This does not equate to a complete suppression of immune response but represents a finely tuned regulation ensuring selective leukocyte passage without compromising BBB integrity [ 25 ]. During certain pathological conditions, leukocytes such as neutrophils and T cells may interact with the BBB in ways that can affect its permeability, through the release of cytokines, reactive oxygen species, and other mediators. However, these interactions do not universally result in BBB disruption and can occur in the absence of significant leakage, depending on the specific disease context and the nature of leukocyte engagement. In the context of neuroinflammatory diseases, such as multiple sclerosis (MS), alterations in the expression and functionality of LAMs have been observed. These changes can facilitate increased adhesion of leukocytes to BECs, potentially leading to BBB disruption under these pathological states [ 26 ]. However, this process reflects a departure from the normative regulatory mechanisms in place under healthy conditions and underscores the complex role of LAMs in both maintaining CNS immunity and contributing to neuroinflammation when dysregulated.
Pericytes is a kind of multi-functional cells embedded within the walls of capillaries throughout the body, including the brain, and play a crucial role in maintaining the integrity of the BBB. They were first identified in the 1870s, and more recently, numerous vascular functions of pericytes have been identified. These include regulation of cerebral blood flow, maintenance of the BBB integrity, and control of vascular development and angiogenesis [ 27 , 28 , 29 ]. In addition to these roles, pericytes have been found to play an active role during neuroinflammation in the adult brain [ 28 , 30 , 31 ]. They can respond differentially, depending on the degree of inflammation, by secreting a set of neurotrophic factors to promote cell survival and regeneration, or by potentiating inflammation through the release of inflammatory mediators (e.g., cytokines and chemokines), and the overexpression of pattern recognition receptors [ 32 ]. In neuroinflammatory conditions like multiple sclerosis, pericytes undergo morphological changes, elongating their processes within inflamed perivascular cuffs [ 33 ]. Exposure to cytokines and extracellular matrix proteins like chondroitin sulfate proteoglycans enhances pericyte secretion of chemokines and promotes macrophage migration [ 33 ]. This implicates pericytes in propagating neuroinflammation through immune cell recruitment. However, pericytes also have neuroprotective capacities dependent on the degree of inflammation [ 34 ]. Through release of neurotrophic factors like BDNF, pericytes can promote neuronal survival and regeneration [ 35 ]. Their expression of cell adhesion molecules likewise facilitates interactions with endothelial cells and astrocytes to maintain cerebrovascular stability [ 36 ]. The dual functionality of pericytes is highlighted by their differential secretome profiles in response to IL-1β [ 37 ]. While pericytes secrete certain pro-inflammatory genes like CCL2 [ 38 ], they also show expression of vascular-stabilized mediators like TIMP3 [ 34 , 39 ]. This nuanced, context-dependent pericyte reactivity fine-tunes neuroinflammatory responses.
Pericyte dysfunction is increasingly recognized as a contributor to the progression of vascular diseases such as stroke and neurodegenerative diseases [ 40 , 41 ]. The therapeutic potential of pericytes to repair cerebral blood vessels and promote angiogenesis due to their ability to possess stem cell-like properties has recently been brought to light. In the context of cerebral blood vessels repair, pericytes can migrate to the site of injury and differentiate into cells that are needed for repair [ 42 , 43 ]. As for promoting angiogenesis, pericytes play a vital role in the formation of new blood vessels from pre-existing ones, a process known as angiogenesis [ 44 ]. They stabilize the newly formed endothelial tubes, modulate blood flow and vascular permeability, and regulate endothelial proliferation, differentiation, migration and survival. However, research has shown that pericytes and endothelial cells have overlapping but distinct secretome profiles in response to IL-1β [ 45 ]. This indicates that these two cell types may respond differently to inflammatory stimuli, which could have implications for understanding how inflammation affects the cerebrovasculature.
In conclusion, pericytes play a critical role in maintaining BBB integrity by controlling various processes. Understanding these mechanisms could provide valuable insights into BBB function and CNS immunity. Future research directions could include exploring the role of other proteins involved in these processes, further investigating the function of pericytes in disease states, and studying how changes in pericyte function could impact BBB integrity and CNS health.
Astrocytes, another critical component of the NVU, also play a crucial role in BBB maintenance. Their end-foot processes enwrap the brain capillaries and provide physical support to the BBB. Through their extensive contact with endothelial cells, astrocytes are vital in the formation and maintenance of the BBB. More specifically, astrocytes can secrete Sonic hedgehog (Shh), thereby stimulating the expression of TJs proteins and junctional adhesion molecule-A (JAM-A) while promoting immune quiescence of BBB by decreasing the expression of chemokines and LAMs [ 46 ]. The Hedgehog (Hh) pathway is involved in embryonic morphogenesis, neuronal guidance, and angiogenesis [ 47 ]. In adult tissues, it participates in vascular proliferation, differentiation, and tissue repair. The Hh pathway provides a barrier-promoting effect and an endogenous anti-inflammatory balance to CNS-directed immune attacks [ 48 ]. In terms of cerebrovascular accidents, astrocytes have been found to play a crucial role in maintaining blood-brain barrier function [ 49 ]. They support neurons and other glia, and react to changes in both the local and external environment. Beyond these homeostatic functions, astrocytes can respond to several stimuli and subsequently display profound genetic, morphological, and functional changes in a process termed reactive astrogliosis.
In addition to their role in maintaining BBB integrity, astrocytes also play a crucial role in controlling water homeostasis in the brain. Aquaporins (AQPs) are transmembrane proteins responsible for fast water movement across cell membranes, including those of astrocytes [ 50 ]. Various subtypes of AQPs (AQP1, AQP3, AQP4, AQP5, AQP8 and AQP9) have been reported to be expressed in astrocytes. The expressions and subcellular localizations of AQPs in astrocytes are highly correlated with both their physiological and pathophysiological functions [ 51 , 52 ].
Basement membrane
The basement membrane (BM) was secreted and maintained by BECs, pericytes and astrocytes. The BM is composed of various proteins, including Laminins, Collagen-IV, Perlecan, and Fibronectin [ 53 , 54 ]. These components interact with each other and with other cells at the BBB. For example, Laminins provide structural support to the BBB and are involved in processes such as cell adhesion, differentiation, migration, and apoptosis, while Collagen-IV provides mechanical strength to the BBB and contributes to its stability [ 53 , 54 ]. Any alterations in these components can lead to BBB dysfunction, which is associated with various neurological disorders. For instance, in conditions such as stroke, the BBB undergoes significant changes, including alterations in the expression of integrins and degradation of surrounding ECM, which indirectly affect the vascular barrier function [ 55 ]. In an animal model of autoimmunity, considerable information has been revealed about the function of different BMs in maintaining BBB integrity and causing neuroinflammation [ 56 , 57 , 58 ].
ECM receptors, such as integrins and dystroglycan, are also expressed at the brain microvasculature and mediate the connections between cellular and matrix components in physiology and disease [ 59 , 60 , 61 ]. These proteins and receptors elicit diverse molecular signals that allow cell adaptation to environmental changes and regulate growth and cell motility [ 62 ].
Microglia and neurons
Finally, microglia and neurons, while not directly forming the BBB, significantly contribute to its function and maintenance. Microglia, the resident immune cells of the brain, play a crucial role in maintaining the integrity of the BBB. Following ischemic stroke, microglia interact with endothelial cells in a paracrine manner to promote angiogenesis and barrier repair [ 63 ]. However, this reparative crosstalk is impeded in aging, implicating declined microglial function in age-related BBB disruption [ 64 ]. Accordingly, experimental depletion of microglia exacerbates injury and edema in aged mice subjected to ischemic stroke, further confirming microglia’s protective influence [ 65 ]. Beyond strokes, microglia also maintain BBB stability in neuroinflammatory conditions by modulating astrocyte reactivity and phagocytosing synapses [ 66 ]. In a model of multiple sclerosis, microglia limited astrocyte activation and protected against BBB leakage, stressing their homeostatic role [ 67 ]. During reactive gliosis induced by stroke, microglia and macrophages preferentially eliminated excitatory synapses while astrocytes cleared inhibitory synapses, preventing excessive imbalance of excitatory/inhibitory tone [ 67 ].These findings underscore diverse mechanisms whereby microglia maintain cerebrovascular equilibrium, ranging from paracrine support of angiogenesis to controlled synapse engulfment. Their protective influence likely declines with aging, permitting BBB hyperpermeability. Boosting microglial function represents a promising strategy to strengthen BBB integrity in neurological disorders.
Neurons also play a significant role in BBB’s function and maintenance. Neurons communicate with other components of the neurovascular unit (NVU), such as endothelial cells, pericytes, and astrocytes, to regulate BBB properties. This communication is also critical for maintaining the CNS homeostasis and for responding to changes in neural activity. In the early postnatal mouse barrel cortex, it was demonstrated that manipulations of sensory inputs resulted in vascular structural changes [ 68 ]. Specifically, this study showed that local sensory-related neural activity promoted the formation of cerebrovascular networks.
Neurovascular coupling refers to the relationship between local neural activity and subsequent changes in cerebral blood flow (CBF) [ 69 ]. The magnitude and spatial location of blood flow changes are tightly linked to changes in neural activity through a complex sequence of coordinated events involving neurons, glia, and vascular cells. This mechanism is crucial as it matches the high energy demand of the brain with a supply of energy substrates from the blood. Evoked the neural activity by high-intensity visual stimulation could drive macroscopic cerebrospinal fluid (CSF) flow in the human brain [ 70 ]. The timing and amplitude of CSF flow were matched to the visually evoked hemodynamic responses, suggesting neural activity can modulate CSF flow via neurovascular coupling.
In addition to their role in neurovascular coupling, neurons also contribute to BBB function through some transporters. It was found that neuronal activity regulates BBB efflux transporter expression and function [ 71 ], which is critical for excluding many small lipophilic molecules from the brain parenchyma. These findings suggest that sensory-related neural activity can influence both vascular structure and BBB function, which could have significant implications for understanding neuro-vascular interaction. An understanding of these intricate structures and their functions is pivotal in comprehending how pathological states, such as obesity, could affect the BBB and ultimately lead to neurological disorders.
Obesity-induced BBB leakage: phenotypes and mechanisms
The impact of obesity on BBB integrity has been an area of increasing scientific scrutiny, given the relationship between obesity and various neurological disorders. The disruption of BBB integrity or non-specific leakage of the BBB, as it is commonly referred to, is a recurrent phenotype observed in obesity, especially in the context of high-fat diet (HFD) intake with a wide duration from 8 to 36 w [ 72 , 73 , 74 , 75 ]. This section seeks to elucidate the molecular mechanisms and phenotypic changes underlying obesity-induced BBB leakage and associated BBB markers of this pathological state.
Changes in tight junctions
A key factor implicated in obesity-induced BBB leakage is the dysregulation of tight junction proteins (TJs). TJs, as earlier stated, are critical in maintaining the restrictive properties of the BBB. However, obesity, particularly under conditions of HFD, can alter the expression and function of these proteins. For instance, Ouyang et al. observed alterations in the expression levels of ZO-1 in microvessels from obese mice modeling by 8 weeks (w) of HFD [ 76 ]. In line with this observation, HFD significantly decreased the protein levels of ZO-1, Claudin-5 and Occludin along with the leakage of brain microvessles after 8 weeks (w) of HFD [ 77 ]. In adult rats, 90 days of high-energy diet (high in saturated fat and glucose) consumption decreased mRNA expression of TJs, particularly Claudin-5 and − 12, in the choroid plexus and the BBB. Consequently, an increased blood-to-brain permeability of sodium fluorescein was observed in the hippocampus [ 78 ]. This underscores the potential for obesity-induced modifications to BBB structure and function.
The adenosine receptor 2a (Adora2a) is increasingly recognized for its significant role in the modulation of neurovascular and neuroinflammatory responses. Activation of Adora2a receptors has been linked to heightened inflammatory responses, contributing to the disruption of the blood-brain barrier (BBB) and subsequent neuronal damage, conditions often exacerbated by obesity and metabolic syndrome. In a rodent model of diet-induced insulin resistance by 16 w of HFD, it was found that chronic activation of Adora2a eroded TJs between BECs, as evidenced by diminished Occludin and Claudin-5 in hippocampal lysates. Considering the detrimental effects associated with Adora2a activation on BBB integrity, antagonism of this receptor presents a promising therapeutic strategy. By inhibiting Adora2a, it is possible to mitigate the receptor-mediated exacerbation of inflammatory processes within the CNS, thereby preserving BBB function and reducing neuroinflammatory sequelae. This premise is supported by several studies demonstrating that Adora2a antagonists can effectively reduce BBB permeability and alleviate inflammatory damage in various neuroinflammatory and neurovascular disorders [ 79 , 80 , 81 ].
In addition, it is noteworthy that obesity not only affects one’s own BBB function, but also has an impact on its offspring. A recent study showed that maternal obesity during pregnancy could impaired BBB formation of the fetal, leading to changes in TJ components of the arcuate nucleus region in offspring’s brain, thereby significantly increasing BBB permeability [ 82 ]. This dysregulation of TJs compromises the integrity of the BBB, increasing its permeability and enabling the passage of potentially harmful substances from the blood into the CNS. Interestingly, recent studies have also linked prolonged HFD intake for 32 w to anxiety-like and depression-like behaviors in mice [ 83 ]. It was found that 24 weeks of HFD consumption induced neurobehavioral deterioration, including increased anxiety-like and depression-like behavior. These behavioral changes were associated with impaired gut microbiota homeostasis and inflammation. Long term HFD may induce certain behavioral phenotypes related to neurological disorders through the gut-brain axis [ 83 ]. In line with this, treatment with the anti-inflammatory molecule palmitoylethanolamide was found to reduce anxiety-like behavior in obese mice modeling by 19 w of HFD, along with dampening systemic and central inflammation [ 84 ]. Taken together, these studies suggest prolonged HFD may not only directly disrupt TJs and BBB integrity through inflammatory and other mechanisms, but also trigger neurobehavioral changes that could secondarily impact BBB function.
Changes in fenestration
As mentioned above, BECs are characterized by a lack of fenestrations, a characteristic that contributes to the high selectivity and restrictive nature of the BBB. Stan et al. identified PV-1 (also known as PLVAP, plasmalemma vesicle-associated protein; or MEGA-32 antigen) as a component of fenestral diaphragms in endothelial cells [ 85 ]. As PV-1 comprises these structures, its regulation could influence fenestration numbers. While PLVAP expressed on fenestrated endothelia and associated with the formation of diaphragms in vesicular structures, it does not serve as a marker for fenestrations within the BBB context. Instead, increases in PLVAP expression may reflect alterations in the molecular composition associated with transcellular pathways rather than the formation of true fenestrae. In a single-cell profiling study, analysis of BECs revealed that among eight major clusters, fenestrated BECs in areas such as the choroid plexus showed the most unique obesity-induced DEGs, where fenestrated endothelia are typical, rather than suggesting the emergence of fenestrations within the BBB due to obesity modeling by 12 w of HFD [ 86 ]. In line with this, Previous study have demonstrated that HFD intake can lead to an increase in endothelial fenestration in the BBB, such as the observed changes in the offspring of gestational obesity in mice [ 82 ]. Worth noticed, these findings, particularly those related to increased permeability in regions like the arcuate nucleus, may reflect localized alterations in BBB properties rather than systemic induction of fenestrations akin to those in inherently fenestrated structures like the choroid plexus.
Therefore, while the effects of HFD on endothelial cell biology are undeniable, the notion that these effects lead to the formation of fenestrations within the typical BBB structure remains debatable. This perspective allows for the possibility that PLVAP-regulated alterations may occur regionally within specific areas of the brain under certain conditions, rather than implying a global change across the entire barrier. The DEGs identified in obesity models, particularly those affecting endothelial cells of the BBB, should be interpreted with an understanding that obesity-induced stress may lead to changes in molecular signaling and barrier properties without necessitating the creation of actual fenestral openings.
Changes in matrix metalloproteinases
Matrix metalloproteinases (MMPs) are a family of endopeptidases that function to degrade and remodel the extracellular matrix (ECM). MMPs are secreted by various cell types including epithelial cells, fibroblasts, and inflammatory cells, playing important roles in physiological processes as well as disease states characterized by tissue damage and inflammation. However, excessive MMPs can lead to pathological ECM degradation and impairment of tissue structure and function, as in the case of blood-brain barrier (BBB) disruption by MMP-2 and MMP-9 [ 87 , 88 , 89 ]. Obesity has been linked to increased expression and activity of certain MMPs, which can impair BBB integrity. For example, plasma MMP-9 levels were found to be elevated in obese subjects and decreased with anti-diabetic treatment [ 90 , 91 ]. Cotemporally, lipocalin-2 was also increased in adipose tissue of obese individuals and correlated with MMP-2 and MMP-9 activity [ 92 ]. MMP-8 levels were similarly increased in obesity and associated with insulin resistance [ 93 ]. Additional studies have assessed levels of MMPs like MMP-2 and MMP-9 along with their inhibitors TIMPs in obese children [ 94 ]. Together, these findings suggest obesity creates a pro-inflammatory state characterized by upregulation of MMPs like MMP-8 and MMP-9, potentially driven by increases in mediators like lipocalin-2. By degrading ECM proteins, these MMPs can impair BBB structural integrity. A general description of obesity-induced BBB leakage was shown in Fig. 2 .
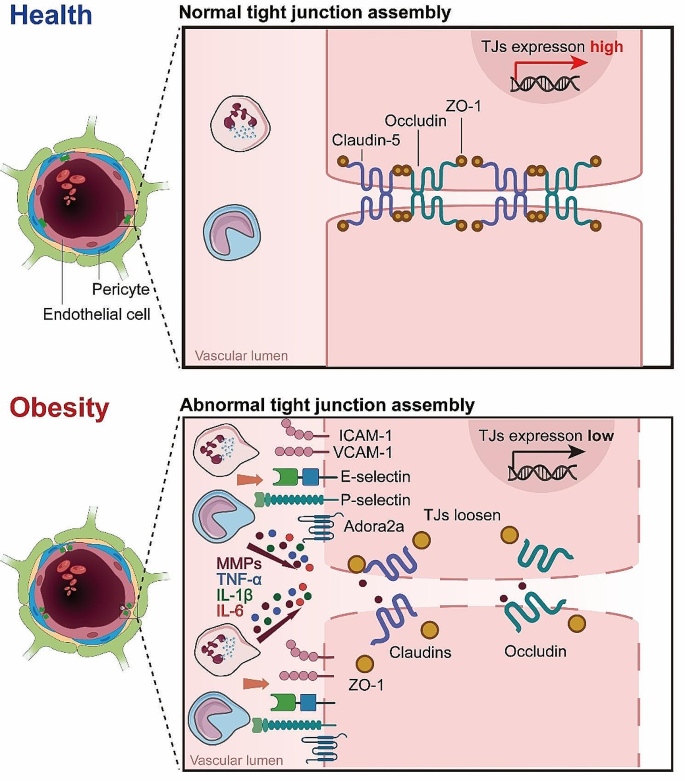
Mechanisms of Obesity-Induced BBB Leakage. Illustration of the complex alterations in the BBB induced by obesity, highlighting the loosening of endothelial cell junctions, increased permeability, and the unusual occurrence of fenestrations. These structural changes are further exacerbated by the activity of MMPs, which degrade extracellular matrix components, facilitating the infiltration of peripheral immune cells, such as neutrophils. This cascade of events underscores the multifaceted and interconnected impact of obesity on BBB integrity and the resulting immune response within the cerebral vasculature
Obesity-induced BBB transport dysfunction: phenotypes and mechanisms
In addition to disrupting the BBB integrity, obesity is associated with alterations in the BBB’s transport functions. These changes not only affect the nutrient supply to the brain but can also influence the entry and clearance of toxins, signaling molecules, and therapeutics. The transport across the BBB is highly regulated, with specific receptors and transporters expressed in BECs mediating the process. However, obesity can induce significant changes in these receptors and transporters, leading to BBB transport dysfunction.
Glucose transport-1 (Glut-1)
One of the key alterations in BBB transport function under obesity conditions is the dysregulation of glucose transport. The primary transporter responsible for glucose transport across the BBB is the glucose transporter 1 (Glut-1), which ensures the supply of glucose from the blood to the brain. However, in the state of obesity, particularly in HFD-induced obesity, the expression and function of Glut-1 have been found to be altered. Specifically, studies in mouse models have demonstrated that acute high-fat feeding for 2–3 days suppressed Glut-1 expression and glucose uptake in the brain [ 95 , 96 ]. Similarly, the mouse insulin resistance model showed that when 2 weeks of HFD significantly downregulated the expression of Glut-1 in the brain, 10 weeks of HFD normalized the expression of Glut-1, suggesting the duration of insulin resistance may influenced the regulation of Glut-1 [ 97 ]. In humans, genetic factors were found to impact Glut-1 levels, which in turn modulates cognitive effects of high-fat intake [ 98 ].
Interestingly, upon more prolonged high-fat feeding for 10 weeks, Glut-1 expression was restored, which was related to the initiation of compensatory mechanisms of vascular endothelial growth factor (VEGF), a key regulator of angiogenesis and vascular function [ 95 ]. Recent studies have illuminated the role of VEGF in respond to metabolic stresses and hypoxic conditions often prevalent in obesity. This response includes the potential to upregulate Glut-1 expression, ostensibly to compensate for altered metabolic demands and ensure sufficient glucose transport across the BBB. However, the interplay between VEGF and Glut-1 in the setting of obesity is multifaceted. While moderate increases in VEGF can be beneficial, aiding in the restoration of Glut-1 levels and maintaining cerebral glucose metabolism, chronic elevations—common in prolonged obesity—may lead to adverse effects [ 95 , 96 ]. Excessive VEGF can contribute to vascular abnormalities and exacerbate BBB disruption, complicating the metabolic landscape of the CNS. Therefore, understanding the dynamic between VEGF-induced Glut-1 modulation and obesity provides insight into the broader implications of metabolic syndrome on BBB health and brain metabolism. This section explores how the nuanced changes in VEGF and Glut-1 expression influenced by obesity underscore the complexity of maintaining BBB integrity and highlight the need for targeted therapeutic strategies to address these metabolic challenges.
Taken together, while short-term high-fat intake appears to impair Glut-1 function and glucose transport at the BBB, compensation may occur with prolonged obesity to normalize Glut-1 expression. However, the brain is the most energy-consuming organ, requiring constant high energy to maintain its function, thereby the temporary disruption in glucose delivery to the brain may be sufficient to impact neuronal health and cognition. Further research into the kinetics of Glut-1 regulation in response to high-fat feeding could delineate the timeframe of BBB transport deficits. This may provide insights into critical windows where impaired brain glucose uptake contributes to neurological disorders associated with obesity.
Alterations in insulin transport have also been observed in obesity, which can profoundly impact neuronal function. Insulin enters the brain via saturation transport and binds to insulin receptors (IR) on BECs, triggering receptor autophosphorylation and downstream signaling cascades involved in glucose uptake, metabolism, neuronal plasticity and survival [ 99 ]. However, studies in obese animal models and human subjects indicate that obesity and HFD feeding can impair insulin transport across the BBB. In preclinical studies using mouse and canine models, HFD feeding for 7 w reduced transport of intravenously injected insulin from the circulation into the brain parenchyma [ 100 , 101 ]. This reduction is associated with central insulin resistance, as evidenced by impaired insulin receptor signaling cascades in the brain from mice subjected to 4 w of HFD [ 102 ]. In human clinical studies, obese subjects were found to have lower cerebrospinal fluid (CSF) insulin levels and attenuated CSF insulin increases after systemic insulin infusion compared to healthy controls [ 103 ]. Together, these animal and human studies demonstrate that obesity disrupts brain endothelial insulin transport, reducing insulin delivery to the CNS. This transport dysfunction contributes to central insulin resistance, a phenomenon often linked with cognitive dysfunction and neurological disorders [ 104 ]. Consistence with this, brain insulin resistance has been recognized as an early characteristic of Alzheimer’s disease [ 105 ]. Elucidating the mechanisms by which high-fat feeding alters insulin receptor expression, trafficking and downstream signaling at the BBB will be critical for developing therapeutic strategies to overcome CNS insulin resistance.
In addition to insulin, the transport of the hormone leptin across the BBB is also altered in obesity. Leptin is also transported into the brain via a saturable transport system and binds to leptin receptors on neurons involved in regulating food intake and energy expenditure. However, multiple studies indicate HFD-induced obesity impairs leptin transport across the BBB. For instance, in obese individuals, the transport level of leptin to the brain is downregulated, as evidenced by a significantly lower ratio of the leptin cerebrospinal fluid (CSF)/serum compared to the healthy lean individuals [ 106 , 107 ]. In obese animal models including rodents subjected to 10 w of HFD and sheep subjected to 40 w of HFD, there is a significant decrease in the rate of leptin transport from blood to brain compared to lean controls [ 108 , 109 , 110 ]. Obesity can inhibit the transport of leptin across the BBB, making it impossible for the brain to receive the “satiety signal” emitted by leptin, leading to overeating and worsening of obesity, which may lead to a series of metabolic diseases. Of notice, with the development of obesity, obese mice modeling by 56 days of HFD respond to leptin for central administration (intracerebroventricularly) rather than peripheral administration (intraperitoneally or subcutaneously) [ 111 , 112 ]. This suggests that. the impairment in leptin transport does not appear to be due to altered leptin receptor expression at the BBB. Rather, transport may be inhibited due to saturation of the carrier system and interactions with other circulating factors, such as the high-level triglycerides [ 113 , 114 , 115 ]. Overall, these findings indicate obese states inhibit leptin’s ability to enter the CNS and bind neuronal targets, despite normal BBB leptin receptor levels. Overcoming the transport block could potentiate leptin’s effects on appetite and weight regulation.
P-glycoprotein (P-gp)
Obesity also affects the function of efflux transporters at the BBB, such as the P-glycoprotein (P-gp). P-gp is an ATP-dependent transporter that functions to pump foreign substances and metabolites out of the brain back into the bloodstream. This helps protect the brain from accumulation of potentially toxic compounds. P-gp is encoded by the ABCB1 gene. A human study found a negative correlation between BMI values and the expression levels of ABCB1 in the brain, suggesting P-gp levels are reduced in obesity [ 116 ]. While the mechanisms linking obesity to P-gp regulation require further elucidation, systemic inflammation appears to play a role. In obese pregnant mice, placental P-gp expression was decreased in tandem with increases in inflammatory cytokines like tumor necrosis factor-alpha (TNF-α), interleukin-1 beta (IL-1β), and interleukin-6 (IL-6) [ 117 ]. Changes in P-gp likely impair efflux of substrates from the brain back into circulation. Overall, although there is limited research on obesity and P-gp, current evidence indicates obesity can suppress P-gp expression and function at the BBB, at least in the obese human. This impairment in a key transporter for xenobiotic clearance may enable accumulation of toxins and drugs in the brain.
L-type amino acid transporter-1 (LAT1)
Amino acid transporters are also affected by obesity. The system L amino acid transporter 1 (LAT1) expressed at the BBB is responsible for transport of large neutral amino acids like leucine into the brain. LAT1 plays a key role in regulating mTORC1 signaling, which controls processes like protein synthesis and autophagy [ 118 ]. Recent studies have found that LAT1 function is altered in obesity models. Mice lacking neuronal LAT1 develop obesity phenotypes including increased adiposity [ 119 ]. LAT1 expression and amino acid uptake are reduced in the hypothalamus of obese, diabetic mice [ 120 ]. In humans, lower expression of the related transporter SLC7A10/ASC-1 in adipose tissue is associated with increased visceral fat, insulin resistance, and adipocyte hypertrophy [ 121 ]. Together, these findings indicate obesity impairs the function of multiple amino acid transporters at the BBB and periphery. This likely dysregulates mTORC1 signaling and other nutrient-sensing pathways, contributing to metabolic dysfunction. The alterations of transporters expressed in endothelial cells was summarized in Fig. 3 .
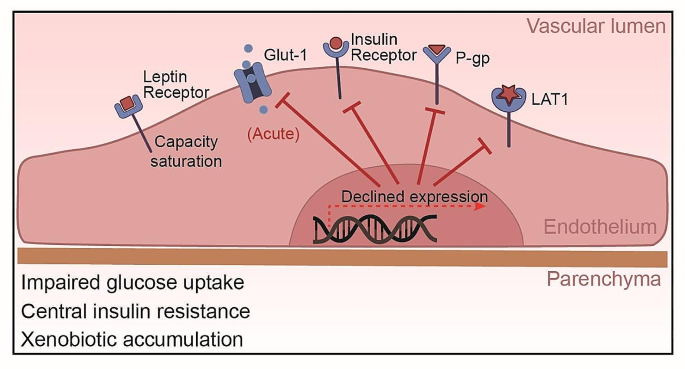
Obesity-Induced Alterations in BBB Transporters. This figure demonstrates the effects of obesity on BBB transporters: Glut-1 function is initially impaired by high-fat intake but may be normalized with prolonged obesity, despite potential impacts on neuronal health and cognition. Brain endothelial insulin transport is disrupted, contributing to central insulin resistance, and often associated with cognitive dysfunction and neurological disorders. Leptin transport impairment is linked to carrier system saturation, independent of leptin receptor expression changes. Obesity suppresses P-glycoprotein expression and function, potentially increasing brain accumulation of toxins and drugs. LAT1 expression and amino acid uptake are reduced in the hypothalamus of obese, diabetic mice, reflecting impaired amino acid transporter function at the BBB.
Evidence of BBB disruption in obesity and type 2 diabetes
Recent evidence has increasingly indicated that metabolic disorders such as obesity and Type 2 Diabetes (T2D) are closely linked to the disruption of the BBB. This section aims to elucidate the relationship between these conditions and BBB integrity, underpinned by recent scientific findings.
BBB Permeability in Type 2 Diabetes: Among the most compelling evidence in this domain is the study conducted by Starr and colleagues [ 122 ], which has demonstrated a significant increase in BBB permeability to gadolinium in patients with well-controlled T2D. This landmark study utilized advanced magnetic resonance imaging techniques to compare BBB integrity between diabetic patients and healthy individuals. The results indicated a pronounced increase in BBB permeability, notably in the basal ganglia region, suggesting a direct impact of T2D on neurovascular integrity. These findings are critical as they highlight the potential for well-controlled T2D to contribute to BBB dysfunction independently of other comorbidities often associated with metabolic syndrome.
The link between obesity and BBB disruption has been further substantiated by studies investigating the levels of specific BBB markers in obese individuals. A longitudinal perspective explores how factors related to adiposity in mid-life can have long-lasting effects on BBB integrity. The study highlights the enduring impact of obesity on neurovascular health, suggesting that the consequences of increased body weight extend far beyond the immediate metabolic disturbances commonly associated with obesity [ 123 ]. Another study focused on Adipsin, a complement system component known to be influenced by adiposity levels, and studied within the cerebrospinal fluid, providing insights into the biochemical pathways through which obesity might mediate changes in BBB integrity. This research suggests a direct link between metabolic health markers and the biochemical status of the BBB, illustrating the intricate connection between systemic metabolic health and neurovascular function [ 124 ]. These studies have shown elevated levels of certain markers, indicating compromised BBB integrity in the context of excessive body weight and associated metabolic derangements. The disruption is believed to be multifactorial, involving mechanisms such as increased systemic inflammation, altered lipid metabolism, and hypertension, all of which are prevalent in obesity and can adversely affect the endothelial cells constituting the BBB.
The mechanistic pathways through which obesity and T2D exacerbate BBB disruption are complex and multifaceted. Inflammatory cytokines, often elevated in obesity and T2D, are known to compromise BBB integrity by altering tight junction protein expression and endothelial cell function. Additionally, the hyperglycemic environment in T2D can induce oxidative stress and microvascular complications, further impairing BBB function. These alterations not only have direct implications for neurovascular health but also predispose individuals to a range of neurological disorders. Takechi et al. [ 125 ] shows that BBB dysfunction precedes cognitive decline and neurodegeneration in a diabetic insulin-resistant mouse modeled by 24 w of high fat and fructose fed, which may imply a causal link. Although this study is more centered around diabetes, the intersection with obesity (through insulin resistance) makes it pertinent. The above findings emphasizing the need for comprehensive management of these metabolic conditions to maintain BBB integrity and overall brain health.
In summary, the accumulating evidence underscores a significant association between obesity, T2D, and BBB disruption. This relationship highlights the importance of managing these metabolic disorders not only for cardiovascular health but also for maintaining the integrity of the neurovascular unit. As research in this field continues to evolve, understanding the specific pathways and impacts will be crucial for developing targeted interventions aimed at preserving BBB integrity in the face of growing obesity and T2D prevalence.
Obesity-induced neuroinflammation: phenotypes and mechanisms
Neuroinflammation, characterized by the activation of resident brain cells (microglia and astrocytes) and infiltration of peripheral immune cells, has been widely recognized as a critical pathological feature of obesity. The increased BBB permeability induced by obesity, as discussed earlier, not only allows harmful substances to penetrate into the CNS but also paves the way for peripheral immune cells to infiltrate the brain. Once in the CNS, these immune cells can instigate an inflammatory response, contributing significantly to obesity-induced neuroinflammation.
Microglia, the primary immune cells of the CNS, show enhanced activation in obesity. Once activated, microglia release a plethora of pro-inflammatory factors, including cytokines like TNF-α, IL-1β, and IL-6, thereby promoting a pro-inflammatory environment within the CNS [ 84 , 126 , 127 , 128 ]. In the research employed bone marrow chimerism mouse subjected to 15 or 30 w of HFD, it was also found that the aggregated inflammatory monocytes/macrophages located in the parenchyma and expressed the microglial marker Iba1 [ 129 ]. Notably, while microglia often exhibit a pro-inflammatory phenotype in the early stages of HFD-induced obesity, their activation state appears to change over time with prolonged exposure. As the study by Baufeld et al. [ 130 ] demonstrated, the initial microglial reaction in the hypothalamus of mice with 3 days of HFD was not accompanied by sustained increased pro-inflammatory cytokines with prolonged 20 w of HFD consumption. Rather, anti-inflammatory genes were upregulated while microglial sensing genes were downregulated [ 130 ]. This indicates that microglia may shift to a more anti-inflammatory or homeostatic phenotype after longer-term HFD consumption.
The regional heterogeneity of microglial responses is another important consideration. Microglia in the hypothalamic arcuate nucleus, for instance, displayed a markedly reaction to 8 weeks of HFD in a region-specific manner [ 130 ]. This underscores how microglia in different brain regions may uniquely adapted to their specific microenvironments and react differently to the metabolic challenges imposed by obesity. Furthermore, the plasticity and ability of microglia to respond to additional stimuli was preserved even after prolonged HFD feeding. When stimulated with LPS ex vivo after 8 weeks of HFD, hypothalamic microglia upregulated inflammatory genes comparable to microglia from control diet mice [ 130 ]. This indicates the microglia retain responsiveness despite adapting to the HFD conditions. Mechanically, Kim et al. [ 131 ] has been instrumental in highlighting the dynamic increase in uncoupling protein 2 ( Ucp2 ) mRNA expression in the hypothalamic microglia of mice following an 8 w-HFD regimen. This increase influences mitochondrial modifications that activate microglia, further contributing to hypothalamic inflammation and the overall susceptibility to obesity. In summary, emerging evidence indicates microglial phenotypes and functions are altered in a temporal and spatial manner by obesity and HFD consumption. While often displaying pro-inflammatory features acutely, microglia may adapt with anti-inflammatory or homeostatic responses over time. Their heterogeneous phenotypes across brain regions and retained ability to respond to stimuli highlight the complexity of microglial reactions in obesity.
Astrocytes, another critical cell type in the CNS, undergo reactive astrogliosis in obesity, characterized by changes in their morphology, proliferation, and function. Similar to microglia, activated astrocytes can also secrete pro-inflammatory cytokines, further exacerbating the neuroinflammatory response. Astrocytes in 16 w-HFD consumption displayed reactive astrogliosis, characterized by altered morphology and upregulation of intermediate filaments like glial fibrillary acidic protein (GFAP) [ 132 ]. This phenotypic shift was observable early during HFD feeding, even preceding substantial weight gain. Lin et al. [ 133 ] elucidates the upregulation of disease-associated astrocyte (DAA) and microglia markers in response to an 12 w-HFD that are similar to the pathogenesis of Alzheimer’s disease. providing a direct link between dietary habits, neuroinflammation, and neurodegeneration.
The functional profile of astrocytes was also altered by obesity-induced astrogliosis. Activated astrocytes upregulate expression of pro-inflammatory cytokines, including IL-6, IL-1β, and TNF-α in mice following an 11 w-HFD regimen [ 134 ]. This creates a self-perpetuating cycle, as increased cytokine levels can further stimulate astrogliosis. Additionally, aberrant release of gliotransmitters like glutamate from reactive astrocytes can also occur, potentially impacting neuronal excitability after a 16 w of HFD [ 126 ].
Multiple signaling pathways have been implicated in driving the astrogliotic transformation of astrocytes in obesity. As Thaler et al. demonstrated, astrocyte-specific inhibition of IKKβ/NF-κB signaling mitigated weight gain, glucose intolerance, and hypothalamic inflammation induced by 11 w of HFD consumption [ 134 ]. This suggests the IKKβ/NF-κB pathway is critical for obesity-related astrogliosis and its metabolic consequences. Calcineurin signaling has also been linked to astrocyte reactivity in response to HFD for 16 w [ 132 ]. Calcineurin inhibition attenuated gliosis in the arcuate nucleus, ventromedial hypothalamus, and dorsomedial hypothalamus of HFD mice. This implicates calcineurin/NFAT as another important mediator of astrocyte activation. In summary, the morphological and functional changes accompanying astrogliosis position astrocytes as key propagators of neuroinflammatory responses in obesity models induced by high-fat feeding. Delineating the intracellular signaling pathways driving these astrocyte alterations, such as IKKβ/NF-κB and calcineurin/NFAT, will contribute to uncover therapeutic targets for mitigating obesity-associated hypothalamic inflammation.
Monocytes/macrophages
Peripheral immune cells, particularly monocytes, have been reported to infiltrate the CNS in obesity. These monocytes can differentiate into macrophages, producing a variety of pro-inflammatory cytokines that exacerbate neuroinflammation. For instance, a bone marrow chimerism mouse model demonstrated that 15 or 30 w of HFD-induced obesity led to a 30% increase of immune cells in the CNS compared to controls [ 129 ]. Most of these cells exhibited a microglia/macrophage phenotype, being CD45 + CD11b + . The ratio of CD11b + CD45 hi to CD11b + CD45 lo cells was elevated, indicating an inflammatory state. In addition to the infiltration, HFD also promotes the differentiation of monocytes into macrophages. It was demonstrated that 14 w of HFD consumption induced the Ly6c high monocytes to differentiate into macrophages in the brain [ 135 ]. Another study showed that prolonged HFD consumption for 4 and 20 w led to expansion of the monocyte-derived macrophage pool in the hypothalamic arcuate nucleus, attributed to enhanced macrophage proliferation [ 136 ]. In mouse models of leptin receptor deficiency, breakdown of the BBB was found to enable macrophage infiltration into the brain of db/db mice [ 137 ]. Leptin resistance, glucose intolerance, and elevated cytokines like IL-1β and TNF-α accompanied the accumulation of macrophages. Notably, this study also suggested that IL-1β potentially play an important role in trafficking of peripheral monocytes into the brain. Overall, these studies indicate HFD-induced obesity facilitates the recruitment and proliferation of macrophages in the brain, propagating inflammation.
While the involvement of monocytes and macrophages in mediating neuroinflammatory responses under obese conditions is evident, it is imperative to scrutinize the methodologies employed in these studies for potential confounding factors. Specifically, investigations utilizing GFP bone marrow chimeras, such as those by Buckman et al. [ 129 ] and Baufeld et al. [ 130 ] mention above, provide critical insights into the trafficking of these immune cells in the context of obesity. However, the integral role of radiation treatment in these experimental designs warrants a careful evaluation of its impact on the observed outcomes. Radiation used to establish bone marrow chimeras, as a preparatory step for tracking immune cell migration, is known to independently alter BBB permeability and trigger pro-inflammatory responses, as detailed by Kierdorf et al. [ 138 ] This raises important considerations for interpreting studies on obesity-associated neuroinflammation: the increased permeability of the BBB and the augmented infiltration of monocytes/macrophages observed could be confounded by the radiation treatment itself, rather than being solely attributable to the effects of obesity.
Acknowledging these potential confounding effects is crucial for a comprehensive understanding of the dynamics between obesity and neurovascular integrity. Future research aimed at elucidating the specific roles of monocytes and macrophages in obesity-induced BBB disruption and brain inflammation should consider employing alternative methodologies that circumvent the need for radiation-induced bone marrow ablation. This approach will ensure a clearer delineation of the direct consequences of obesity on neuroimmune interactions and BBB integrity, free from the complicating effects of experimental interventions.
By carefully considering these methodological nuances, we can enhance our understanding of the complex interplay between obesity, BBB integrity, and the role of monocytes/macrophages in neuroinflammation, thereby paving the way for more targeted and effective therapeutic strategies.
Pro-inflammatory factors
Obesity-induced neuroinflammation is also marked by elevated expression levels of pro-inflammatory factors. In addition to TNF-α, IL-1β, and IL-6, other pro-inflammatory molecules such as inducible nitric oxide synthase (iNOS) and cyclooxygenase-2 (COX-2) show increased expression in obesity. These molecules, produced by both infiltrating peripheral immune cells and activated resident brain cells, not only propagate inflammation but can also contribute to BBB disruption and neuronal damage. In human frontal cortex, it was reported that increased BMI has been found to cause iNOS-mediated inflammatory activity [ 139 ]. A recent finding demonstrated that iNOS promotes hypothalamic insulin resistance in obese rats. Aberrant nitrosative stress such as S-nitrosatiion (also referred to as S-nitrosylation) is closely associate with various neurological disorders such as AD and Parkinson’s Disease (PD) [ 140 , 141 ]. Inhibition of central iNOS ameliorated not only glucose metabolism, but also macrophage activation induced inflammation in hypothalamus of HFD-induced obesity mice [ 136 ]. In addition, 18 or 20 w of HFD consumption also can significantly enhance the expression of COX-2 in the hippocampus of the mice [ 142 , 143 ]. An increased activity of COX-2-PEG2 signaling pathway has been considered to play a key role in impairing hippocampal neuronal function and cognition [ 144 , 145 ].
Additionally, the aberrant activation of the inflammasome complexes, especially NOD-like receptor thermal protein domain associated protein 3 (NLRP3), plays a vital role in obesity-induced neuroinflammatory [ 146 ]. Within the CNS, microglia accumulate lipid droplets and activate NLRP3 inflammasomes under hyperglycemic conditions due to impaired lipophagy [ 147 ]. The microglial specific inflammatory amplifier TREM1 (triggering receptor expressed on myeloid cells), resulting in the buildup of microglial TREM1, was found to aggravates the HG-induced lipophagy damage and subsequently promoted HG-induced neuroinflammatory cascades via NLRP3 (NLR family pyrin domain containing 3) inflammasome. Pharmacological blockade of TREM1 with LP17 in db/db mice and HFD/STZ mice inhibited accumulation of lipid droplets and TREM1, reduced hippocampal neuronal inflammatory damage, and consequently improved cognitive functions [ 147 ].
Together, these diverse peripheral and central inflammatory pathways contribute to BBB disruption, neurotoxicity, and cognitive deficits associated with obesity. Targeting shared processes like nitrosative stress, which interacts with iNOS signaling, may simultaneously mitigate obesity-related neuroinflammation. The upregulation of pivotal inflammatory enzymes like iNOS and COX-2 highlights the multi-faceted nature of obesity-induced inflammation and the need for strategies that address key underlying pathways.
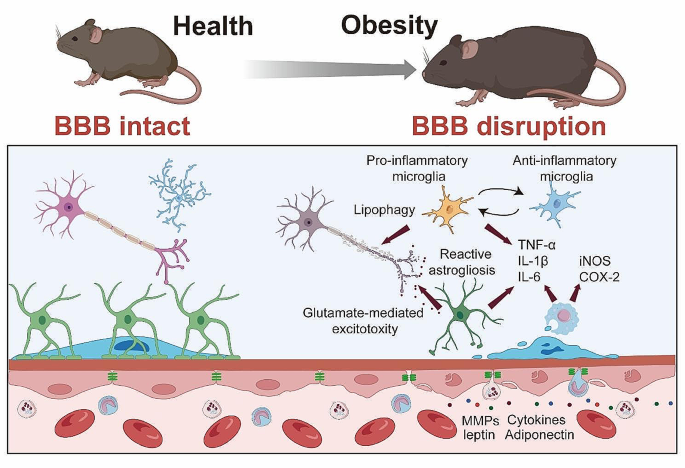
Mechanisms of Obesity-Induced Neuroinflammation . Enhanced microglial activation in obesity leads to increased release of pro-inflammatory cytokines (TNF-α, IL-1β, IL-6), contributing to a pro-inflammatory CNS environment. Concurrently, obesity triggers reactive astrogliosis in astrocytes characterized by morphological and functional changes, and secretion of pro-inflammatory cytokines. The infiltration of peripheral monocytes into the CNS, and differentiation into macrophages further exacerbates neuroinflammation through additional cytokine production. Elevated expression of pro-inflammatory factors such as iNOS and COX-2 in obesity are also depicted, highlighting their potential role in neuroinflammation, BBB disruption, and neuronal damage
Dietary influences on BBB integrity and obesity outcomes
The intricate relationship between obesity and inflammation underscores the multifaceted nature of BBB dysfunction. While the systemic inflammation associated with obesity undoubtedly impacts BBB integrity, it is imperative to address the direct and indirect effects of dietary patterns on neurovascular health. The link between diet, obesity, and BBB integrity provides a unique lens through which to understand the broader implications of nutritional habits on cerebral health and disease susceptibility. Freeman et al. [ 148 ] highlights the adverse effects of a 6 month consumption of high-fat/high-cholesterol (HFHC) diet on BBB function, demonstrating a reduction in BBB integrity markers and an increase in inflammatory responses within the hippocampus in middle-aged rats. This suggests that HFHC diets, beyond contributing to obesity, may directly compromise the BBB, thereby facilitating a neuroinflammatory state that could underpin cognitive decline. Similarly, Davidson et al. [ 149 ] reported on the effects of high-energy diets on hippocampal-dependent cognitive functions and BBB integrity. Their findings emphasized that diets high in saturated fats and sugars, hallmarks of the “Western” diet, not only contribute to obesity but also impair types of learning and memory dependent on the hippocampus. The unique aspect of this research is the differentiation between diet-induced obese and diet-resistant rats, highlighting how diet affects cognitive function and BBB integrity differently based on individual susceptibility to obesity. This is particularly concerning as it implies that dietary components can directly influence cognitive functions by altering hippocampal integrity and possibly disrupting the BBB. The dietary components and direct influences on BBB was detailed in Table 1 , which underscores the potential for dietary modifications as preventive or mitigative strategies against obesity-induced neurovascular alterations.
Emerging therapeutic strategies in tackling obesity-induced BBB dysfunction
In addressing the complexities of obesity-induced metabolic syndrome and its impact on the central nervous system, a spectrum of experimental therapies is currently under investigation, each targeting distinct pathological mechanisms. One promising approach involves the decrease of BBB permeability. Agents such as Palmitoylethanolamide, Topiramate, and Nicotine have demonstrated potential in modulating BBB dynamics, suggesting a pivotal role in mitigating obesity-related neurological sequelae in mice subjected to 10–36 w of HFD or 10 w of high saturated fatty acids diet consumption [ 72 , 73 , 84 ]. Among these, Palmitoylethanolamide has shown promise, not only in attenuating anxiety-like behavior but also in modulating neurotransmitter levels such as dopamine turnover and γ-aminobutyric acid (GABA) levels in the amygdala from mice subjected to 19 w of HFD consumption [ 84 ]. Additionally, it has been found to reduce systemic inflammation markers like TNF-α and IL-1β, attenuate hypothalamic injury, and decrease neuroinflammation and BBB permeability in the hippocampus. However, while its effects on BBB permeability are notable, further investigation is necessary to fully understand its long-term efficacy and safety profile, particularly in relation to chronic administration and potential systemic effects.
Topiramate, another therapeutic agent, has been demonstrated its capability to decrease BBB permeability in mice subjected to 13 and 36 w of HFD [ 72 ]. It achieves this through increasing the expression of tight junction proteins like ZO-1 and Claudin-12, which are crucial in maintaining BBB integrity. Topiramate also exhibits properties that inhibit oxidative stress, a common pathological feature in obesity-related neurological disorders. In this regard, agents like Dapsone and Resveratrol have garnered attention for their potential to protect tight junctions and reduce BBB disruption in mice following 8 w of HFD [ 74 , 77 ]. Dapsone, for instance, has been found to decrease brain microvascular leakage, which is often exacerbated in obesity. It does so by inhibiting the oxidation of low-density lipoproteins (LDL), a process that is detrimental to the BBB [ 77 ]. Additionally, Dapsone has shown efficacy in protecting tight junction proteins such as ZO-1, Claudin-5, and Occludin, further enhancing its role in preserving BBB integrity in mice following 8 w of HFD. Similarly, Resveratrol, a naturally occurring polyphenolic compound, has been demonstrated its capacity to fortify BBB tight junctions. Its neuroprotective properties extend beyond just maintaining the BBB; Resveratrol also exhibits antioxidative and anti-inflammatory effects, which are beneficial in addressing the multifactorial aspects of obesity-induced neural damage in mice subjected 8 w of HFD [ 74 ]. However, while these agents show promise, their clinical application faces challenges. The variability in individual responses and potential side effects, such as hypersensitivity reactions with Dapsone, require careful consideration. Future research must focus on optimizing these therapies, possibly through targeted delivery systems or combination therapies, to enhance their protective effects on the BBB while minimizing adverse reactions. Despite these benefits, the challenge with Topiramate lies in its potential side effects, such as cognitive disturbances and weight loss, which might limit its use in certain patient populations. Future research should focus on optimizing its dosage and delivery mechanisms to maximize its therapeutic benefits while minimizing adverse effects. Besides the aforementioned strategies, other pharmacological effects pivotal in managing obesity-induced complications include the modulation of oxidative stress and cellular death, the regulation of metabolic pathways, and the enhancement of neural regeneration, each contributing uniquely towards mitigating the multifaceted challenges posed by obesity.
In conclusion, the emerging therapeutic strategies discussed in this section underscore the complexity and multidimensionality of tackling obesity-induced metabolic syndrome and its neurological implications. From enhancing BBB integrity to addressing oxidative stress, metabolic dysregulation, and promoting neuroregeneration, each approach offers a unique angle in combating the extensive impact of obesity [ 150 , 151 ]. This multifaceted approach not only broadens our understanding but also paves the way for innovative and comprehensive treatments in the ongoing battle against obesity-related neurological disorders. The major experimental therapies are summarized in Table 2 , which presents a curated list of compounds demonstrated to exert protective effects against the adverse consequences of obesity on the brain, particularly focusing on maintaining or restoring BBB integrity. Each compound included has been selected based on empirical evidence from studies highlighting its efficacy in counteracting obesity-induced neurovascular dysfunction.
Conclusion and future directions
The intricate association between obesity and the BBB has been the primary focus of this review. We have delved into the fundamental understanding of BBB dysfunction in the context of obesity, detailing the altered BBB permeability, transport dysfunction, and neuroinflammation, each contributing to a multifaceted pathophysiological landscape that paves the way for obesity-related neurological disorders. Despite the progress made, several gaps persist in our understanding of obesity-induced BBB dysfunction. For instance, more in-depth studies on the temporal relationship between obesity and BBB dysfunction could provide insight into the initial triggers of these changes. Furthermore, it remains unclear whether BBB dysfunction is a universal feature of obesity or if it varies with factors such as the degree and duration of obesity, age, sex, and genetic predisposition. Moreover, the potential reversibility of obesity-induced BBB changes and the optimal strategies for achieving such reversibility warrant exploration.
Recognizing BBB dysfunction’s role in obesity-related neurological diseases holds significant promise for future therapeutic advancements. By better understanding the interactions between BBB dysfunction and neurodegeneration, we may discover new strategies to mitigate or even prevent the deleterious effects of obesity on the CNS. There lies immense potential in targeting the BBB for therapeutic intervention, with the promise of not only alleviating obesity-induced BBB dysfunction but also mitigating its downstream effects, including neuroinflammation and neurodegeneration.
In conclusion, obesity-induced BBB dysfunction represents an area of research with implications extending beyond the realm of obesity to a broad spectrum of neurological disorders. A more comprehensive understanding of obesity’s influence on the CNS would ultimately benefit those affected by obesity and its neurological consequences.
Data availability
No datasets were generated or analysed during the current study.
WHO. Obesity and overweight. 2022.
Pedditzi E, Peters R, Beckett N. The risk of overweight/obesity in mid-life and late life for the development of dementia: a systematic review and meta-analysis of longitudinal studies. Age Ageing. 2016;45:14–21.
Article PubMed Google Scholar
Flores-Cordero JA, Perez-Perez A, Jimenez-Cortegana C, Alba G, Flores-Barragan A, Sanchez-Margalet V. Obesity as a Risk Factor for Dementia and Alzheimer’s Disease: The Role of Leptin. Int J Mol Sci 2022, 23.
Wieckowska-Gacek A, Mietelska-Porowska A, Wydrych M, Wojda U. Western diet as a trigger of Alzheimer’s disease: from metabolic syndrome and systemic inflammation to neuroinflammation and neurodegeneration. Ageing Res Rev. 2021;70:101397.
Article CAS PubMed Google Scholar
Haley MJ, Lawrence CB. Obesity and stroke: can we translate from rodents to patients? J Cereb Blood Flow Metab. 2016;36:2007–21.
Article CAS PubMed PubMed Central Google Scholar
Lee SH, Jung JM, Park MH. Obesity paradox and stroke outcomes according to stroke subtype: a propensity score-matched analysis. Int J Obes (Lond). 2023;47:669–76.
Akyea RK, Doehner W, Iyen B, Weng SF, Qureshi N, Ntaios G. Obesity and long-term outcomes after incident stroke: a prospective population-based cohort study. J Cachexia Sarcopenia Muscle. 2021;12:2111–21.
Article PubMed PubMed Central Google Scholar
Mi Y, Qi G, Vitali F, Shang Y, Raikes AC, Wang T, Jin Y, Brinton RD, Gu H, Yin F. Loss of fatty acid degradation by astrocytic mitochondria triggers neuroinflammation and neurodegeneration. Nat Metab. 2023;5:445–65.
Morant-Ferrando B, Jimenez-Blasco D, Alonso-Batan P, Agulla J, Lapresa R, Garcia-Rodriguez D, Yunta-Sanchez S, Lopez-Fabuel I, Fernandez E, Carmeliet P, et al. Fatty acid oxidation organizes mitochondrial supercomplexes to sustain astrocytic ROS and cognition. Nat Metab. 2023;5:1290–302.
Profaci CP, Munji RN, Pulido RS, Daneman R. The blood-brain barrier in health and disease: important unanswered questions. J Exp Med 2020, 217.
Knox EG, Aburto MR, Clarke G, Cryan JF, O’Driscoll CM. The blood-brain barrier in aging and neurodegeneration. Mol Psychiatry. 2022;27:2659–73.
Schaeffer S, Iadecola C. Revisiting the neurovascular unit. Nat Neurosci. 2021;24:1198–209.
Sweeney MD, Zhao Z, Montagne A, Nelson AR, Zlokovic BV. Blood-brain barrier: from physiology to Disease and back. Physiol Rev. 2019;99:21–78.
Langen UH, Ayloo S, Gu C. Development and Cell Biology of the blood-brain barrier. Annu Rev Cell Dev Biol. 2019;35:591–613.
Huang X, Hussain B, Chang J. Peripheral inflammation and blood-brain barrier disruption: effects and mechanisms. CNS Neurosci Ther. 2021;27:36–47.
Paik DT, Tian L, Williams IM, Rhee S, Zhang H, Liu C, Mishra R, Wu SM, Red-Horse K, Wu JC. Single-cell RNA sequencing unveils Unique Transcriptomic signatures of Organ-Specific endothelial cells. Circulation. 2020;142:1848–62.
Nguyen YTK, Ha HTT, Nguyen TH, Nguyen LN. The role of SLC transporters for brain health and disease. Cell Mol Life Sci. 2021;79:20.
Chai AB, Callaghan R, Gelissen IC. Regulation of P-Glycoprotein in the brain. Int J Mol Sci 2022, 23.
Cui Y, Wang Y, Song X, Ning H, Zhang Y, Teng Y, Wang J, Yang X. Brain endothelial PTEN/AKT/NEDD4-2/MFSD2A axis regulates blood-brain barrier permeability. Cell Rep. 2021;36:109327.
Andreone BJ, Chow BW, Tata A, Lacoste B, Ben-Zvi A, Bullock K, Deik AA, Ginty DD, Clish CB, Gu CH. Blood-brain barrier permeability is regulated by lipid transport-dependent suppression of Caveolae-Mediated Transcytosis. Neuron. 2017;94:581–.
Ayloo S, Gu C. Transcytosis at the blood-brain barrier. Curr Opin Neurobiol. 2019;57:32–8.
Ben-Zvi A, Lacoste B, Kur E, Andreone BJ, Mayshar Y, Yan H, Gu C. Mfsd2a is critical for the formation and function of the blood-brain barrier. Nature. 2014;509:507–11.
Yang YR, Xiong XY, Liu J, Wu LR, Zhong Q, Zhou K, Meng ZY, Liu L, Wang FX, Gong QW et al. Mfsd2a (Major Facilitator Superfamily Domain Containing 2a) attenuates Intracerebral Hemorrhage-Induced blood-brain barrier disruption by inhibiting vesicular transcytosis. J Am Heart Assoc 2017, 6.
Chapman PT, Haskard DO. Leukocyte adhesion molecules. Br Med Bull. 1995;51:296–311.
Rossler K, Neuchrist C, Kitz K, Scheiner O, Kraft D, Lassmann H. Expression of leucocyte adhesion molecules at the human blood-brain barrier (BBB). J Neurosci Res. 1992;31:365–74.
Allavena R, Noy S, Andrews M, Pullen N. CNS elevation of vascular and not mucosal addressin cell adhesion molecules in patients with multiple sclerosis. Am J Pathol. 2010;176:556–62.
Armulik A, Genove G, Mae M, Nisancioglu MH, Wallgard E, Niaudet C, He L, Norlin J, Lindblom P, Strittmatter K, et al. Pericytes regulate the blood-brain barrier. Nature. 2010;468:557–61.
Longden TA, Zhao G, Hariharan A, Lederer WJ. Pericytes and the Control of Blood Flow in Brain and Heart. Annu Rev Physiol. 2023;85:137–64.
Hall CN, Reynell C, Gesslein B, Hamilton NB, Mishra A, Sutherland BA, O’Farrell FM, Buchan AM, Lauritzen M, Attwell D. Capillary pericytes regulate cerebral blood flow in health and disease. Nature. 2014;508:55–60.
Bell RD, Winkler EA, Sagare AP, Singh I, LaRue B, Deane R, Zlokovic BV. Pericytes control key neurovascular functions and neuronal phenotype in the adult brain and during brain aging. Neuron. 2010;68:409–27.
Sweeney MD, Ayyadurai S, Zlokovic BV. Pericytes of the neurovascular unit: key functions and signaling pathways. Nat Neurosci. 2016;19:771–83.
Nyul-Toth A, Kozma M, Nagyoszi P, Nagy K, Fazakas C, Hasko J, Molnar K, Farkas AE, Vegh AG, Varo G, et al. Expression of pattern recognition receptors and activation of the non-canonical inflammasome pathway in brain pericytes. Brain Behav Immun. 2017;64:220–31.
Kaushik DK, Bhattacharya A, Lozinski BM, Wee Yong V. Pericytes as mediators of infiltration of macrophages in multiple sclerosis. J Neuroinflammation. 2021;18:301.
Medina-Flores F, Hurtado-Alvarado G, Deli MA, Gomez-Gonzalez B. The active role of Pericytes during Neuroinflammation in the adult brain. Cell Mol Neurobiol. 2023;43:525–41.
Arimura K, Ago T, Kamouchi M, Nakamura K, Ishitsuka K, Kuroda J, Sugimori H, Ooboshi H, Sasaki T, Kitazono T. PDGF receptor beta signaling in pericytes following ischemic brain injury. Curr Neurovasc Res. 2012;9:1–9.
Shimizu F, Sano Y, Saito K, Abe MA, Maeda T, Haruki H, Kanda T. Pericyte-derived glial cell line-derived neurotrophic factor increase the expression of claudin-5 in the blood-brain barrier and the blood-nerve barrier. Neurochem Res. 2012;37:401–9.
Dave JM, Mirabella T, Weatherbee SD, Greif DM. Pericyte ALK5/TIMP3 Axis contributes to endothelial morphogenesis in the developing brain. Dev Cell. 2018;44:665–e678666.
Duan L, Zhang XD, Miao WY, Sun YJ, Xiong G, Wu Q, Li G, Yang P, Yu H, Li H, et al. PDGFRbeta cells rapidly relay Inflammatory Signal from the Circulatory System to neurons via chemokine CCL2. Neuron. 2018;100:183–e200188.
Schrimpf C, Koppen T, Duffield JS, Boer U, David S, Ziegler W, Haverich A, Teebken OE, Wilhelmi M. TIMP3 is regulated by Pericytes upon Shear stress detection leading to a modified endothelial cell response. Eur J Vasc Endovasc Surg. 2017;54:524–33.
Cai W, Liu H, Zhao J, Chen LY, Chen J, Lu Z, Hu X. Pericytes in Brain Injury and Repair after ischemic stroke. Transl Stroke Res. 2017;8:107–21.
Lendahl U, Nilsson P, Betsholtz C. Emerging links between cerebrovascular and neurodegenerative diseases-a special role for pericytes. EMBO Rep. 2019;20:e48070.
Sakuma R, Kawahara M, Nakano-Doi A, Takahashi A, Tanaka Y, Narita A, Kuwahara-Otani S, Hayakawa T, Yagi H, Matsuyama T, Nakagomi T. Brain pericytes serve as microglia-generating multipotent vascular stem cells following ischemic stroke. J Neuroinflammation. 2016;13:57.
Nakata M, Nakagomi T, Maeda M, Nakano-Doi A, Momota Y, Matsuyama T. Induction of Perivascular neural stem cells and possible contribution to Neurogenesis following transient brain Ischemia/Reperfusion Injury. Transl Stroke Res. 2017;8:131–43.
Caporarello N, D’Angeli F, Cambria MT, Candido S, Giallongo C, Salmeri M, Lombardo C, Longo A, Giurdanella G, Anfuso CD, Lupo G. Pericytes in Microvessels: from mural function to brain and retina regeneration. Int J Mol Sci 2019, 20.
Smyth LCD, Rustenhoven J, Park TI, Schweder P, Jansson D, Heppner PA, O’Carroll SJ, Mee EW, Faull RLM, Curtis M, Dragunow M. Unique and shared inflammatory profiles of human brain endothelia and pericytes. J Neuroinflammation. 2018;15:138.
Alvarez JI, Dodelet-Devillers A, Kebir H, Ifergan I, Fabre PJ, Terouz S, Sabbagh M, Wosik K, Bourbonniere L, Bernard M, et al. The hedgehog pathway promotes blood-brain barrier integrity and CNS immune quiescence. Science. 2011;334:1727–31.
Hill SA, Fu M, Garcia ADR. Sonic hedgehog signaling in astrocytes. Cell Mol Life Sci. 2021;78:1393–403.
Xie Y, Kuan AT, Wang W, Herbert ZT, Mosto O, Olukoya O, Adam M, Vu S, Kim M, Tran D, et al. Astrocyte-neuron crosstalk through hedgehog signaling mediates cortical synapse development. Cell Rep. 2022;38:110416.
Song S, Huang H, Guan X, Fiesler V, Bhuiyan MIH, Liu R, Jalali S, Hasan MN, Tai AK, Chattopadhyay A, et al. Activation of endothelial Wnt/beta-catenin signaling by protective astrocytes repairs BBB damage in ischemic stroke. Prog Neurobiol. 2021;199:101963.
Zhou Z, Zhan J, Cai Q, Xu F, Chai R, Lam K, Luan Z, Zhou G, Tsang S, Kipp M et al. The water transport system in astrocytes-aquaporins. Cells 2022, 11.
Verkman AS, Smith AJ, Phuan PW, Tradtrantip L, Anderson MO. The aquaporin-4 water channel as a potential drug target in neurological disorders. Expert Opin Ther Targets. 2017;21:1161–70.
Chai RC, Jiang JH, Wong AY, Jiang F, Gao K, Vatcher G. Hoi Yu AC: AQP5 is differentially regulated in astrocytes during metabolic and traumatic injuries. Glia. 2013;61:1748–65.
Xu L, Nirwane A, Yao Y. Basement membrane and blood-brain barrier. Stroke Vasc Neurol. 2019;4:78–82.
Baeten KM, Akassoglou K. Extracellular matrix and matrix receptors in blood-brain barrier formation and stroke. Dev Neurobiol. 2011;71:1018–39.
Nirwane A, Yao Y. Laminins and their receptors in the CNS. Biol Rev Camb Philos Soc. 2019;94:283–306.
Wu C, Ivars F, Anderson P, Hallmann R, Vestweber D, Nilsson P, Robenek H, Tryggvason K, Song J, Korpos E, et al. Endothelial basement membrane laminin alpha5 selectively inhibits T lymphocyte extravasation into the brain. Nat Med. 2009;15:519–27.
Zhang X, Wang Y, Song J, Gerwien H, Chuquisana O, Chashchina A, Denz C, Sorokin L. The endothelial basement membrane acts as a checkpoint for entry of pathogenic T cells into the brain. J Exp Med 2020, 217.
Sixt M, Engelhardt B, Pausch F, Hallmann R, Wendler O, Sorokin LM. Endothelial cell laminin isoforms, laminins 8 and 10, play decisive roles in T cell recruitment across the blood-brain barrier in experimental autoimmune encephalomyelitis. J Cell Biol. 2001;153:933–46.
Wu X, Reddy DS. Integrins as receptor targets for neurological disorders. Pharmacol Ther. 2012;134:68–81.
Lilja J, Ivaska J. Integrin activity in neuronal connectivity. J Cell Sci 2018, 131.
Park YK, Goda Y. Integrins in synapse regulation. Nat Rev Neurosci. 2016;17:745–56.
Krishnaswamy VR, Benbenishty A, Blinder P, Sagi I. Demystifying the extracellular matrix and its proteolytic remodeling in the brain: structural and functional insights. Cell Mol Life Sci. 2019;76:3229–48.
Jin C, Shi Y, Shi L, Leak RK, Zhang W, Chen K, Ye Q, Hassan S, Lyu J, Hu X, et al. Leveraging single-cell RNA sequencing to unravel the impact of aging on stroke recovery mechanisms in mice. Proc Natl Acad Sci U S A. 2023;120:e2300012120.
Li X, Li Y, Jin Y, Zhang Y, Wu J, Xu Z, Huang Y, Cai L, Gao S, Liu T, et al. Transcriptional and epigenetic decoding of the microglial aging process. Nat Aging. 2023;3:1288–311.
Marino Lee S, Hudobenko J, McCullough LD, Chauhan A. Microglia depletion increase brain injury after acute ischemic stroke in aged mice. Exp Neurol. 2021;336:113530.
Liddelow SA, Guttenplan KA, Clarke LE, Bennett FC, Bohlen CJ, Schirmer L, Bennett ML, Munch AE, Chung WS, Peterson TC, et al. Neurotoxic reactive astrocytes are induced by activated microglia. Nature. 2017;541:481–7.
Shi X, Luo L, Wang J, Shen H, Li Y, Mamtilahun M, Liu C, Shi R, Lee JH, Tian H, et al. Stroke subtype-dependent synapse elimination by reactive gliosis in mice. Nat Commun. 2021;12:6943.
Gour A, Boergens KM, Heike N, Hua Y, Laserstein P, Song K, Helmstaedter M. Postnatal connectomic development of inhibition in mouse barrel cortex. Science 2021, 371.
Kaplan L, Chow BW, Gu C. Neuronal regulation of the blood-brain barrier and neurovascular coupling. Nat Rev Neurosci. 2020;21:416–32.
Williams SD, Setzer B, Fultz NE, Valdiviezo Z, Tacugue N, Diamandis Z, Lewis LD. Correction: neural activity induced by sensory stimulation can drive large-scale cerebrospinal fluid flow during wakefulness in humans. PLoS Biol. 2023;21:e3002123.
Pulido RS, Munji RN, Chan TC, Quirk CR, Weiner GA, Weger BD, Rossi MJ, Elmsaouri S, Malfavon M, Deng A, et al. Neuronal activity regulates blood-brain barrier Efflux Transport through endothelial circadian genes. Neuron. 2020;108:937–e952937.
Salameh TS, Mortell WG, Logsdon AF, Butterfield DA, Banks WA. Disruption of the hippocampal and hypothalamic blood-brain barrier in a diet-induced obese model of type II diabetes: prevention and treatment by the mitochondrial carbonic anhydrase inhibitor, topiramate. Fluids Barriers CNS. 2019;16:1.
Elahy M, Lam V, Pallebage-Gamarallage MM, Giles C, Mamo JC, Takechi R. Nicotine attenuates disruption of blood-brain Barrier Induced by Saturated-Fat Feeding in Wild-Type mice. Nicotine Tob Res. 2015;17:1436–41.
Chang HC, Tai YT, Cherng YG, Lin JW, Liu SH, Chen TL, Chen RM. Resveratrol attenuates high-fat diet-induced disruption of the blood-brain barrier and protects brain neurons from apoptotic insults. J Agric Food Chem. 2014;62:3466–75.
Takechi R, Pallebage-Gamarallage MM, Lam V, Giles C, Mamo JC. Nutraceutical agents with anti-inflammatory properties prevent dietary saturated-fat induced disturbances in blood-brain barrier function in wild-type mice. J Neuroinflammation. 2013;10:73.
Ouyang S, Hsuchou H, Kastin AJ, Wang Y, Yu C, Pan W. Diet-induced obesity suppresses expression of many proteins at the blood-brain barrier. J Cereb Blood Flow Metab. 2014;34:43–51.
Zhan R, Zhao M, Zhou T, Chen Y, Yu W, Zhao L, Zhang T, Wang H, Yang H, Jin Y, et al. Dapsone protects brain microvascular integrity from high-fat diet induced LDL oxidation. Cell Death Dis. 2018;9:683.
Kanoski SE, Zhang Y, Zheng W, Davidson TL. The effects of a high-energy diet on hippocampal function and blood-brain barrier integrity in the rat. J Alzheimers Dis. 2010;21:207–19.
Yamamoto M, Guo DH, Hernandez CM, Stranahan AM. Endothelial Adora2a activation promotes blood-brain barrier breakdown and cognitive impairment in mice with Diet-Induced insulin resistance. J Neurosci. 2019;39:4179–92.
Zhang Y, Shen L, Xie J, Li L, Xi W, Li B, Bai Y, Yao H, Zhang S, Han B. Pushen capsule treatment promotes functional recovery after ischemic stroke. Phytomedicine. 2023;111:154664.
Zhou Y, Zeng X, Li G, Yang Q, Xu J, Zhang M, Mao X, Cao Y, Wang L, Xu Y, et al. Inactivation of endothelial adenosine A(2A) receptors protects mice from cerebral ischaemia-induced brain injury. Br J Pharmacol. 2019;176:2250–63.
Kim DW, Glendining KA, Grattan DR, Jasoni CL. Maternal obesity in the mouse compromises the blood-brain barrier in the Arcuate Nucleus of offspring. Endocrinology. 2016;157:2229–42.
Wu H, Zhang W, Huang M, Lin X, Chiou J. Prolonged high-Fat Diet Consumption throughout Adulthood in mice Induced Neurobehavioral Deterioration via Gut-Brain Axis. Nutrients 2023, 15.
Lama A, Pirozzi C, Severi I, Morgese MG, Senzacqua M, Annunziata C, Comella F, Del Piano F, Schiavone S, Petrosino S, et al. Palmitoylethanolamide dampens neuroinflammation and anxiety-like behavior in obese mice. Brain Behav Immun. 2022;102:110–23.
Stan RV, Kubitza M, Palade GE. PV-1 is a component of the fenestral and stomatal diaphragms in fenestrated endothelia. Proc Natl Acad Sci U S A. 1999;96:13203–7.
Bondareva O, Rodriguez-Aguilera JR, Oliveira F, Liao L, Rose A, Gupta A, Singh K, Geier F, Schuster J, Boeckel JN, et al. Single-cell profiling of vascular endothelial cells reveals progressive organ-specific vulnerabilities during obesity. Nat Metab. 2022;4:1591–610.
Barr TL, Latour LL, Lee KY, Schaewe TJ, Luby M, Chang GS, El-Zammar Z, Alam S, Hallenbeck JM, Kidwell CS, Warach S. Blood-brain barrier disruption in humans is independently associated with increased matrix metalloproteinase-9. Stroke. 2010;41:e123–128.
Palus M, Zampachova E, Elsterova J, Ruzek D. Serum matrix metalloproteinase-9 and tissue inhibitor of metalloproteinase-1 levels in patients with tick-borne encephalitis. J Infect. 2014;68:165–9.
Dal-Pizzol F, Rojas HA, dos Santos EM, Vuolo F, Constantino L, Feier G, Pasquali M, Comim CM, Petronilho F, Gelain DP, et al. Matrix metalloproteinase-2 and metalloproteinase-9 activities are associated with blood-brain barrier dysfunction in an animal model of severe sepsis. Mol Neurobiol. 2013;48:62–70.
Dandona P, Ghanim H, Monte SV, Caruana JA, Green K, Abuaysheh S, Lohano T, Schentag J, Dhindsa S, Chaudhuri A. Increase in the mediators of asthma in obesity and obesity with type 2 diabetes: reduction with weight loss. Obes (Silver Spring). 2014;22:356–62.
Article CAS Google Scholar
Unal R, Yao-Borengasser A, Varma V, Rasouli N, Labbate C, Kern PA, Ranganathan G. Matrix metalloproteinase-9 is increased in obese subjects and decreases in response to pioglitazone. J Clin Endocrinol Metab. 2010;95:2993–3001.
Catalan V, Gomez-Ambrosi J, Rodriguez A, Ramirez B, Silva C, Rotellar F, Gil MJ, Cienfuegos JA, Salvador J, Fruhbeck G. Increased adipose tissue expression of lipocalin-2 in obesity is related to inflammation and matrix metalloproteinase-2 and metalloproteinase-9 activities in humans. J Mol Med (Berl). 2009;87:803–13.
Lauhio A, Farkkila E, Pietilainen KH, Astrom P, Winkelmann A, Tervahartiala T, Pirila E, Rissanen A, Kaprio J, Sorsa TA, Salo T. Association of MMP-8 with obesity, smoking and insulin resistance. Eur J Clin Invest. 2016;46:757–65.
Belo VA, Souza-Costa DC, Lana CM, Caputo FL, Marcaccini AM, Gerlach RF, Bastos MG, Tanus-Santos JE. Assessment of matrix metalloproteinase (MMP)-2, MMP-8, MMP-9, and their inhibitors, the tissue inhibitors of metalloproteinase (TIMP)-1 and TIMP-2 in obese children and adolescents. Clin Biochem. 2009;42:984–90.
Jais A, Solas M, Backes H, Chaurasia B, Kleinridders A, Theurich S, Mauer J, Steculorum SM, Hampel B, Goldau J, et al. Myeloid-cell-derived VEGF maintains brain glucose uptake and limits cognitive impairment in obesity. Cell. 2016;165:882–95.
Haley MJ, Krishnan S, Burrows D, de Hoog L, Thakrar J, Schiessl I, Allan SM, Lawrence CB. Acute high-fat feeding leads to disruptions in glucose homeostasis and worsens stroke outcome. J Cereb Blood Flow Metab. 2019;39:1026–37.
Ogata S, Ito S, Masuda T, Ohtsuki S. Changes of blood-brain barrier and brain parenchymal protein expression levels of mice under different insulin-resistance conditions Induced by High-Fat Diet. Pharm Res. 2019;36:141.
Schuler R, Seebeck N, Osterhoff MA, Witte V, Floel A, Busjahn A, Jais A, Bruning JC, Frahnow T, Kabisch S, et al. VEGF and GLUT1 are highly heritable, inversely correlated and affected by dietary fat intake: consequences for cognitive function in humans. Mol Metab. 2018;11:129–36.
Banks WA, Owen JB, Erickson MA. Insulin in the brain: there and back again. Pharmacol Ther. 2012;136:82–93.
Kaiyala KJ, Prigeon RL, Kahn SE, Woods SC, Schwartz MW. Obesity induced by a high-fat diet is associated with reduced brain insulin transport in dogs. Diabetes. 2000;49:1525–33.
Urayama A, Banks WA. Starvation and triglycerides reverse the obesity-induced impairment of insulin transport at the blood-brain barrier. Endocrinology. 2008;149:3592–7.
Gray SM, Aylor KW, Barrett EJ. Unravelling the regulation of insulin transport across the brain endothelial cell. Diabetologia. 2017;60:1512–21.
Kern W, Benedict C, Schultes B, Plohr F, Moser A, Born J, Fehm HL, Hallschmid M. Low cerebrospinal fluid insulin levels in obese humans. Diabetologia. 2006;49:2790–2.
Kothari V, Luo Y, Tornabene T, O’Neill AM, Greene MW, Geetha T, Babu JR. High fat diet induces brain insulin resistance and cognitive impairment in mice. Biochim Biophys Acta Mol Basis Dis. 2017;1863:499–508.
Talbot K, Wang HY, Kazi H, Han LY, Bakshi KP, Stucky A, Fuino RL, Kawaguchi KR, Samoyedny AJ, Wilson RS, et al. Demonstrated brain insulin resistance in Alzheimer’s disease patients is associated with IGF-1 resistance, IRS-1 dysregulation, and cognitive decline. J Clin Invest. 2012;122:1316–38.
Caro JF, Kolaczynski JW, Nyce MR, Ohannesian JP, Opentanova I, Goldman WH, Lynn RB, Zhang PL, Sinha MK, Considine RV. Decreased cerebrospinal-fluid/serum leptin ratio in obesity: a possible mechanism for leptin resistance. Lancet. 1996;348:159–61.
Schwartz MW, Peskind E, Raskind M, Boyko EJ, Porte D Jr. Cerebrospinal fluid leptin levels: relationship to plasma levels and to adiposity in humans. Nat Med. 1996;2:589–93.
Burguera B, Couce ME, Curran GL, Jensen MD, Lloyd RV, Cleary MP, Poduslo JF. Obesity is associated with a decreased leptin transport across the blood-brain barrier in rats. Diabetes. 2000;49:1219–23.
Adam CL, Findlay PA. Decreased blood-brain leptin transfer in an ovine model of obesity and weight loss: resolving the cause of leptin resistance. Int J Obes (Lond). 2010;34:980–8.
Banks WA, Farrell CL. Impaired transport of leptin across the blood-brain barrier in obesity is acquired and reversible. Am J Physiol Endocrinol Metab. 2003;285:E10–15.
Van Heek M, Compton DS, France CF, Tedesco RP, Fawzi AB, Graziano MP, Sybertz EJ, Strader CD, Davis HR Jr. Diet-induced obese mice develop peripheral, but not central, resistance to leptin. J Clin Invest. 1997;99:385–90.
Halaas JL, Boozer C, Blair-West J, Fidahusein N, Denton DA, Friedman JM. Physiological response to long-term peripheral and central leptin infusion in lean and obese mice. Proc Natl Acad Sci U S A. 1997;94:8878–83.
Banks WA, Niehoff ML, Martin D, Farrell CL. Leptin transport across the blood-brain barrier of the Koletsky rat is not mediated by a product of the leptin receptor gene. Brain Res. 2002;950:130–6.
Banks WA, Coon AB, Robinson SM, Moinuddin A, Shultz JM, Nakaoke R, Morley JE. Triglycerides induce leptin resistance at the blood-brain barrier. Diabetes. 2004;53:1253–60.
Banks WA, Farr SA, Salameh TS, Niehoff ML, Rhea EM, Morley JE, Hanson AJ, Hansen KM, Craft S. Triglycerides cross the blood-brain barrier and induce central leptin and insulin receptor resistance. Int J Obes (Lond). 2018;42:391–7.
Vendelbo J, Olesen RH, Lauridsen JK, Rungby J, Kleinman JE, Hyde TM, Larsen A. Increasing BMI is associated with reduced expression of P-glycoprotein (ABCB1 gene) in the human brain with a stronger association in African americans than caucasians. Pharmacogenomics J. 2018;18:121–6.
Wang C, Li H, Luo C, Li Y, Zhang Y, Yun D, Mu D, Zhou K, Hua Y. The effect of maternal obesity on the expression and functionality of placental P-glycoprotein: implications in the individualized transplacental digoxin treatment for fetal heart failure. Placenta. 2015;36:1138–47.
Cormerais Y, Vucetic M, Parks SK, Pouyssegur J. Amino acid transporters are a vital focal point in the control of mTORC1 Signaling and Cancer. Int J Mol Sci 2020, 22.
Park G, Fukasawa K, Horie T, Masuo Y, Inaba Y, Tatsuno T, Yamada T, Tokumura K, Iwahashi S, Iezaki T et al. l-Type amino acid transporter 1 in hypothalamic neurons in mice maintains energy and bone homeostasis. JCI Insight 2023, 8.
Jersin RA, Jonassen LR, Dankel SN. The neutral amino acid transporter SLC7A10 in adipose tissue, obesity and insulin resistance. Front Cell Dev Biol. 2022;10:974338.
Jersin RA, Tallapragada DSP, Madsen A, Skartveit L, Fjaere E, McCann A, Lawrence-Archer L, Willems A, Bjune JI, Bjune MS, et al. Role of the neutral amino acid transporter SLC7A10 in adipocyte lipid storage, obesity, and insulin resistance. Diabetes. 2021;70:680–95.
Starr JM, Wardlaw J, Ferguson K, MacLullich A, Deary IJ, Marshall I. Increased blood-brain barrier permeability in type II diabetes demonstrated by gadolinium magnetic resonance imaging. J Neurol Neurosurg Psychiatry. 2003;74:70–6.
Gustafson DR, Karlsson C, Skoog I, Rosengren L, Lissner L, Blennow K. Mid-life adiposity factors relate to blood-brain barrier integrity in late life. J Intern Med. 2007;262:643–50.
Schmid A, Hochberg A, Berghoff M, Schlegel J, Karrasch T, Kaps M, Schaffler A. Quantification and regulation of adipsin in human cerebrospinal fluid (CSF). Clin Endocrinol (Oxf). 2016;84:194–202.
Takechi R, Lam V, Brook E, Giles C, Fimognari N, Mooranian A, Al-Salami H, Coulson SH, Nesbit M, Mamo JCL. Blood-brain barrier dysfunction precedes cognitive decline and Neurodegeneration in Diabetic insulin resistant mouse model: an implication for Causal Link. Front Aging Neurosci. 2017;9:399.
Nerurkar PV, Johns LM, Buesa LM, Kipyakwai G, Volper E, Sato R, Shah P, Feher D, Williams PG, Nerurkar VR. Momordica charantia (bitter melon) attenuates high-fat diet-associated oxidative stress and neuroinflammation. J Neuroinflammation. 2011;8:64.
Yi CX, Tschop MH, Woods SC, Hofmann SM. High-fat-diet exposure induces IgG accumulation in hypothalamic microglia. Dis Model Mech. 2012;5:686–90.
CAS PubMed PubMed Central Google Scholar
Sundaram K, Mu J, Kumar A, Behera J, Lei C, Sriwastva MK, Xu F, Dryden GW, Zhang L, Chen S, et al. Garlic exosome-like nanoparticles reverse high-fat diet induced obesity via the gut/brain axis. Theranostics. 2022;12:1220–46.
Buckman LB, Hasty AH, Flaherty DK, Buckman CT, Thompson MM, Matlock BK, Weller K, Ellacott KL. Obesity induced by a high-fat diet is associated with increased immune cell entry into the central nervous system. Brain Behav Immun. 2014;35:33–42.
Baufeld C, Osterloh A, Prokop S, Miller KR, Heppner FL. High-fat diet-induced brain region-specific phenotypic spectrum of CNS resident microglia. Acta Neuropathol. 2016;132:361–75.
Kim JD, Yoon NA, Jin S, Diano S. Microglial UCP2 mediates inflammation and obesity Induced by High-Fat Feeding. Cell Metab. 2019;30:952–e962955.
Pfuhlmann K, Schriever SC, Legutko B, Baumann P, Harrison L, Kabra DG, Baumgart EV, Tschop MH, Garcia-Caceres C, Pfluger PT. Calcineurin a beta deficiency ameliorates HFD-induced hypothalamic astrocytosis in mice. J Neuroinflammation. 2018;15:35.
Lin L, Basu R, Chatterjee D, Templin AT, Flak JN, Johnson TS. Disease-associated astrocytes and microglia markers are upregulated in mice fed high fat diet. Sci Rep. 2023;13:12919.
Douglass JD, Dorfman MD, Fasnacht R, Shaffer LD, Thaler JP. Astrocyte IKKbeta/NF-kappaB signaling is required for diet-induced obesity and hypothalamic inflammation. Mol Metab. 2017;6:366–73.
Lee AG, Kang S, Im S, Pak YK. Cinnamic acid attenuates peripheral and hypothalamic inflammation in High-Fat Diet-Induced obese mice. Pharmaceutics 2022, 14.
Lee CH, Kim HJ, Lee YS, Kang GM, Lim HS, Lee SH, Song DK, Kwon O, Hwang I, Son M, et al. Hypothalamic macrophage inducible nitric oxide synthase mediates obesity-Associated Hypothalamic inflammation. Cell Rep. 2018;25:934–e946935.
Stranahan AM, Hao S, Dey A, Yu X, Baban B. Blood-brain barrier breakdown promotes macrophage infiltration and cognitive impairment in leptin receptor-deficient mice. J Cereb Blood Flow Metab. 2016;36:2108–21.
Kierdorf K, Katzmarski N, Haas CA, Prinz M. Bone marrow cell recruitment to the brain in the absence of irradiation or parabiosis bias. PLoS ONE. 2013;8:e58544.
Lauridsen JK, Olesen RH, Vendelbo J, Hyde TM, Kleinman JE, Bibby BM, Brock B, Rungby J, Larsen A. High BMI levels associate with reduced mRNA expression of IL10 and increased mRNA expression of iNOS (NOS2) in human frontal cortex. Transl Psychiatry. 2017;7:e1044.
Nakamura T, Tu S, Akhtar MW, Sunico CR, Okamoto S, Lipton SA. Aberrant protein s-nitrosylation in neurodegenerative diseases. Neuron. 2013;78:596–614.
Nakamura T, Lipton SA. Protein S-Nitrosylation as a therapeutic target for neurodegenerative diseases. Trends Pharmacol Sci. 2016;37:73–84.
de Paiva IHR, da Silva RS, Mendonca IP, Duarte-Silva E, Botelho de Souza JR, Peixoto CA. Fructooligosaccharide (FOS) and Galactooligosaccharide (GOS) improve Neuroinflammation and Cognition by Up-regulating IRS/PI3K/AKT signaling pathway in Diet-induced obese mice. J Neuroimmune Pharmacol. 2023;18:427–47.
Terzo S, Calvi P, Nuzzo D, Picone P, Allegra M, Mule F, Amato A. Long-Term Ingestion of Sicilian Black Bee Chestnut Honey and/or D-Limonene counteracts brain damage Induced by High Fat-Diet in obese mice. Int J Mol Sci 2023, 24.
Zhu X, Yao Y, Yang J, Zhengxie J, Li X, Hu S, Zhang A, Dong J, Zhang C, Gan G. COX-2-PGE(2) signaling pathway contributes to hippocampal neuronal injury and cognitive impairment in PTZ-kindled epilepsy mice. Int Immunopharmacol. 2020;87:106801.
Sil S, Ghosh T. Role of cox-2 mediated neuroinflammation on the neurodegeneration and cognitive impairments in colchicine induced rat model of Alzheimer’s Disease. J Neuroimmunol. 2016;291:115–24.
Litwiniuk A, Bik W, Kalisz M, Baranowska-Bik A. Inflammasome NLRP3 Potentially Links Obesity-Associated Low-Grade Systemic Inflammation and Insulin Resistance with Alzheimer’s Disease. Int J Mol Sci 2021, 22.
Li Q, Zhao Y, Guo H, Li Q, Yan C, Li Y, He S, Wang N, Wang Q. Impaired lipophagy induced-microglial lipid droplets accumulation contributes to the buildup of TREM1 in diabetes-associated cognitive impairment. Autophagy. 2023;19:2639–56.
Freeman LR, Granholm AC. Vascular changes in rat hippocampus following a high saturated fat and cholesterol diet. J Cereb Blood Flow Metab. 2012;32:643–53.
Davidson TL, Monnot A, Neal AU, Martin AA, Horton JJ, Zheng W. The effects of a high-energy diet on hippocampal-dependent discrimination performance and blood-brain barrier integrity differ for diet-induced obese and diet-resistant rats. Physiol Behav. 2012;107:26–33.
Wang Q, Yuan J, Yu Z, Lin L, Jiang Y, Cao Z, Zhuang P, Whalen MJ, Song B, Wang XJ, et al. FGF21 attenuates high-Fat Diet-Induced Cognitive Impairment via Metabolic Regulation and anti-inflammation of obese mice. Mol Neurobiol. 2018;55:4702–17.
Li S, Liang T, Zhang Y, Huang K, Yang S, Lv H, Chen Y, Zhang C, Guan X. Vitexin alleviates high-fat diet induced brain oxidative stress and inflammation via anti-oxidant, anti-inflammatory and gut microbiota modulating properties. Free Radic Biol Med. 2021;171:332–44.
Download references
This work was supported by the National Natural Science Foundation of China (32170985, 82273923), National Key Research and Development Program of China (2023YFE0202200 and 2021YFA0910000), Guangdong Province Basic and Applied Basic Research Grant (2021B1515120089), Shenzhen Science and Technology Program (JCYJ20210324115800003, ZDSYS20190902093409851 JCYJ20220531100203008), International collaboration project of Chinese Academy of Sciences (172644KYSB20200045), CAS-Croucher Funding Scheme for Joint Laboratories, and Guangdong Innovation Platform of Translational Research for Cerebrovascular Diseases.
Author information
Authors and affiliations.
Key Laboratory of Biomedical Imaging Science, Shenzhen Institute of Advanced Technology, System of Chinese Academy of Sciences, Chinese Academy of Sciences, Shenzhen, Guangdong, China
Ziying Feng, Cheng Fang, Yinzhong Ma & Junlei Chang
University of Chinese Academy of Sciences, Beijing, China
Ziying Feng & Junlei Chang
Institute of Biomedicine and Biotechnology, Shenzhen Institute of Advanced Technology, Chinese Academy of Sciences, Xueyuan Ave 1068, Nanshan, Shenzhen, 518055, Guangdong, China
Yinzhong Ma & Junlei Chang
You can also search for this author in PubMed Google Scholar
Contributions
Ziying Feng and Yinzhong Ma wrote the main manuscript text and created the table and figures. Cheng Fang participated in the literature review and data summary. Junlei Chang was the guarantor and revised the manuscript. All authors reviewed the final manuscript.
Corresponding authors
Correspondence to Yinzhong Ma or Junlei Chang .
Ethics declarations
Ethics approval and consent to participate.
Not applicable. This manuscript is a review article and does not involve any new studies of human or animal subjects performed by any of the authors.
Competing interests
The authors declare no competing interests.
Additional information
Publisher’s note.
Springer Nature remains neutral with regard to jurisdictional claims in published maps and institutional affiliations.
Rights and permissions
Open Access This article is licensed under a Creative Commons Attribution 4.0 International License, which permits use, sharing, adaptation, distribution and reproduction in any medium or format, as long as you give appropriate credit to the original author(s) and the source, provide a link to the Creative Commons licence, and indicate if changes were made. The images or other third party material in this article are included in the article’s Creative Commons licence, unless indicated otherwise in a credit line to the material. If material is not included in the article’s Creative Commons licence and your intended use is not permitted by statutory regulation or exceeds the permitted use, you will need to obtain permission directly from the copyright holder. To view a copy of this licence, visit http://creativecommons.org/licenses/by/4.0/ . The Creative Commons Public Domain Dedication waiver ( http://creativecommons.org/publicdomain/zero/1.0/ ) applies to the data made available in this article, unless otherwise stated in a credit line to the data.
Reprints and permissions
About this article
Cite this article.
Feng, Z., Fang, C., Ma, Y. et al. Obesity-induced blood-brain barrier dysfunction: phenotypes and mechanisms. J Neuroinflammation 21 , 110 (2024). https://doi.org/10.1186/s12974-024-03104-9
Download citation
Received : 31 January 2024
Accepted : 17 April 2024
Published : 27 April 2024
DOI : https://doi.org/10.1186/s12974-024-03104-9
Share this article
Anyone you share the following link with will be able to read this content:
Sorry, a shareable link is not currently available for this article.
Provided by the Springer Nature SharedIt content-sharing initiative
- Blood-brain barrier
- Neuroinflammation
- Vascular permeability
- Neurological disorders
Journal of Neuroinflammation
ISSN: 1742-2094
- Submission enquiries: [email protected]

IMAGES
VIDEO
COMMENTS
The Blue Brain Project's Blue Gene is a 4-rack system that has 4,096 nodes, equal to 8,192 CPUs, with a peak performance of 22.4 TFLOPS. A 64-rack machine should provide 180 TFLOPS, or 360 TFLOPS ...
The Blue Brain Project carries immense significance as it endeavors to unravel these mysteries. By simulating the brain's structure and function, this project holds the potential to revolutionize our understanding of neurological disorders, inform the development of novel therapies, and shed light on the nature of consciousness itself [2].
II. LITERATURE REVIEW Since it began in 2005, the Blue Brain Project has been the subject of a lot of studies and publications. The following papers and articles about the Blue Brain Project are noteworthy: 1. "A Digital Reconstruction of the Neocortical Microcircuitry" is a landmark work by Markram et al. (2015)
T he brain weighs about 1,500 grams (3 pounds) and constitutes. about 2 percent of total body weig ht. It consists of three major divisions; The massive paired hemispheres of the cerebrum. The ...
This study has made an attempt to review critically review the concept of Blue Brain. Index Terms Blue Brain, artificial brain, Nanobots. ... [RECHZIG'15] - 28 August 2015 th Artificial Intelligence-A Literature Survey of Blue Brain 1 MRS.SHANMUGAPRIYA, 2K.KAVERI , 3M. MUTHUSELVI,4 S.PEREZHILI 1 ASSISTANT PROFESSOR, 234UG SCHOLAR, DEPARTMENT ...
A Literature Review on Blue Brain. N. Chauhan, Gaurav Singhania, Amit Pandey. Published 2019. Philosophy. Human Brain is the most complicated creature on earth that will be demolished after certain decay of years. This research paper is based on the theoritical concept -"How its intelligence can be preserved for future use and what ...
Abstract: "Blue brain" is the first virtual brain in the world. It is a computer that functions similarly to the human brain. The Blue Brain Project began developing the neocortical pillar in July 2005 in collaboration with EPFL (Ecole Polytechnique Fdrale de Lausanne), Professor Henry Markram of the Brain Mind Institute, and IBM (International Business Machines).
This paper presents a literature survey on the Blue Brain technology that promptly answers all these sparkling questions. Scientists today are in research to take artificial intelligence to a level beyond everything, to create a virtual brain that could think, react, make decisions and also keep everything in some form of memory to do ...
The Blue Brain project simulates the human brain by gathering data from its surroundings using specialized software, analyzing it using neuronal electrophysiology and morphology, and recreating it on computers so that after the body has perished, the digitally generated brain will function as the thinking process. Abstract: God's most significant and unique creation is the human brain.
The blue brain is a way of dealing with the world. 2. The stored knowledge and memory in blue brain can sustain for a long period of time. 3. Review of activities, meetings, information to be provided, etc. These are exceptionally huge capabilities that a smart machine can achieve reliably. 4.
The Blue Brain Project. It is argued that the time is right to begin assimilating the wealth of data that has been accumulated over the past century and start building biologically accurate models of the brain from first principles to aid the understanding of brain function and dysfunction. Expand.
2015. TLDR. A literature survey on the Blue Brain technology, which promptly answers all these sparkling questions about how to model a brain and upload the contents of the real natural brain into it thereby propagating life even after death. Expand. 5.
Blue Brain stands as the world's first virtual brain, a revolutionary concept seeking to replicate the cognitive abilities of the human brain through artificial intelligence. The essence of the Blue Brain project lies in the creation of a virtual brain that continues to function even after an individual's physical demise. This virtual brain ...
Blue brain is the name of the world's first virtual brain, basically an artificial brain. ... ―A Review on Blue Brain Technology‖, I nternational J ournal of Trend in Research and Develo pment ...
rack (1,024 nodes = 2,048 CPUs) therefore has 2.8 teraFLOPS or TFLOPS, and a peak of 5.6 TFLOPS. The Blue Brain. Projects Blue Gene is a 4-rack system that has 4,096 nodes, equal to 8,192 CPUs ...
A Review on Blue Brain Technology. 1 Kavya Priya G.V and 2 Monika Sruthi.J, 1,2 IT Department, Sri Krishna Arts and Science College, Coimbatore, Tamil Nadu, India. Abstract: Human brain is the ...
Abstract: Human brain is the most valuable creation. The man is intelligent because of the brain. "Blue brain" is the name of the world's first virtual brain. That means a machine can function as human brain. Today scientists are in research to create an artificial brain that can think, response, take decision, and keep anything in memory.
the name "Blue Brain". 2.2 NEED FOR THE BLUE BRAIN: The scientists have moved towards the invention of blue brain due to two important facts are: 1. Some people have a brain to great extends. After their death we would lost their knowledge and intelligence. Using this concept, we can still use their
Blue Brain Search and Blue Graph enabled Blue Brain scientists to read, analyze and synthesize the knowledge contained in over 240'000 open access scientific papers available in the CORD-19 dataset v47. The Knowledge Graph guided review of the COVID-19-related literature performed using Blue Brain Search and Blue Graph enabled
Blue Brain technology aims to create a virtual brain at cellular level to develop and acquire knowledge of our brain and enable quick treatment of brain related disease. This project was begun by a scientist at EPEL, Switzerland named Henry Markram. On 2005, IBM [International Business Machines] and EPEL [Ecole polytechnique federale] launched ...
Light is a fundamental aspect of our lives, being involved in the regulation of numerous processes in our body. While blue light has always existed in nature, with the ever-growing number of electronic devices that make use of short wavelength (blue) light, the human retina has seen increased exposure to it. Because it is at the high-energy end ...
Literature Review of Blue Brain - Free download as PDF File (.pdf), Text File (.txt) or read online for free. literature review of blue brain
In this narrative, comprehensive, and updated review of the literature, we summarize evidence about the effectiveness of interventions aimed at reducing emotion dysregulation and improving emotion regulation in children, adolescents, and adults. After introducing emotion dysregulation and emotion regulation from a theoretical standpoint, we discuss the factors commonly associated with emotion ...
Hyperserotonemia is one of the most studied endophenotypes in autism spectrum disorder (ASD), but there are still no unequivocal results about its causes or biological and behavioral outcomes. This systematic review summarizes the studies investigating the relationship between blood serotonin (5-HT) levels and ASD, comparing diagnostic tools, analytical methods, and clinical outcomes. A ...
Sep 2022. Dhana Sony .J. Dr Sundar Ganesh C S. R. Bhavani. N Sathiyanathan. PDF | On Jun 15, 2018, Mannu Kumari and others published Review paper on Blue Brain Technology | Find, read and cite all ...
Obesity, a burgeoning global health issue, is increasingly recognized for its detrimental effects on the central nervous system, particularly concerning the integrity of the blood-brain barrier (BBB). This manuscript delves into the intricate relationship between obesity and BBB dysfunction, elucidating the underlying phenotypes and molecular mechanisms. We commence with an overview of the BBB ...